- 1School of Earth and Environment, University of Leeds, Leeds, United Kingdom
- 2Petriva Ltd., University of Leeds, Leeds, United Kingdom
The Earth’s subsurface not only provides a wide range of natural resources but also contains large pore volume that can be used for storing both anthropogenic waste and energy. For example, geothermal energy may be extracted from hot water contained or injected into deep reservoirs and disused coal mines; CO2 may be stored within depleted petroleum reservoirs and deep saline aquifers; nuclear waste may be disposed of within mechanically stable impermeable strata; surplus heat may be stored within shallow aquifers or disused coal mines. Using the subsurface in a safe manner requires a fundamental understanding of the physiochemical processes which occur when decarbonising technologies are implemented and operated. Here, thermal, hydrological, mechanical and chemical perturbations and their dynamics need to be considered. Consequently, geoscience will play a central role in Society’s quest to reduce greenhouse gas emissions. This contribution provides a review of the physiochemical processes related to key technologies that utilize the subsurface for reducing greenhouse gas emissions and the resultant challenges associated with these technologies. Dynamic links between the geomechanical, geochemical and hydrological processes differ between technologies and the geology of the locations in which such technologies are deployed. We particularly focus on processes occurring within the lithologies most commonly considered for decarbonisation technologies. Therefore, we provide a brief comparison between the lithologies, highlighting the main advantages and disadvantages of each, and provide a list of key parameters and properties which have first order effects on the performance of specific rock types, and consequently should be considered during reservoir evaluation for decarbonising technology installation. The review identifies several key knowledge gaps that need to be filled to improve reservoir evaluation and performance prediction to be able to utilize the subsurface efficiently and sustainably. Most importantly, the biggest uncertainties emerge in prediction of fracture pattern development and understanding the extent and timescales of chemical reactions that occur within the decarbonising applications where external fluid or gas is cyclically injected and invariably causes disequilibrium within the system. Furthermore, it is clear that whilst geoscience can show us the opportunities to decarbonise our cities and industries, an interdisciplinary approach is needed to realize these opportunities, also involving social science, end-users and stakeholders.
Introduction
Human reliance on carbon-intensive energy sources is overwhelmingly accepted to be accelerating climate change (IPCC, 2013), which is likely to have severe consequences unless global warming is limited to 1.5°C above pre-industrial levels in accordance with the Paris Agreement (IPCC, Forthcoming 2018). Rapid decarbonisation, resulting in net-zero or sub-zero greenhouse gas (GHG) emissions, is necessary to meet this goal. The rate at which we reduce GHG emissions will be driven by economic factors, social acceptance, technological advances as well as political will. These factors are highly dependent on the details of the individual technology or the set of technologies to be employed. Many decarbonisation technologies are directly linked to the subsurface; examples are Compressed Air Energy Storage (CAES), hydrogen storage, geothermal heat and energy extraction, Underground Thermal Energy Storage (UTES), as well as technologies that will play a crucial role in energy transition such as Carbon Capture and Storage (CCS) and radioactive waste disposal as a necessary part in nuclear power generation (Figure 1). Consequently, processes occurring in the subsurface are key to the suitability and cost of the specific decarbonising technology as well as way it is implemented. Engineered solutions are highly dependent on the characteristics of the subsurface, where during exploration, implementation and utilization, geomechanical, geochemical and geobiological processes may alter the subsurface potentially impacting economics and safety. The most fundamental task for the geological community to support geological decarbonisation technologies is to characterize the geochemical and geomechanical nature of the subsurface because only specific lithologies and their properties are suitable (Stephenson et al., 2019). A second common challenge is the need to understand better the flow of fluids in the deep subsurface, whether that may be water, steam, hydrogen, CO2, or petroleum. The presence of several immiscible fluid phases in the subsurface, reactive rock-fluid interfaces, permeability variations through time and space as well as rock, stress and pore pressure heterogeneity makes this task particularly difficult. Taking the complexity of the system into account requires in-depth knowledge of the dynamics between interrelated thermal-hydrological-mechanical-chemical (THMC) processes over large time-scales. For example, reactive transport models, which at present mainly focus on the chemical reactions associated with fluid influx and/or outflow, need linking to simultaneous changes in the physical and mechanical properties of a storage or energy extraction site.
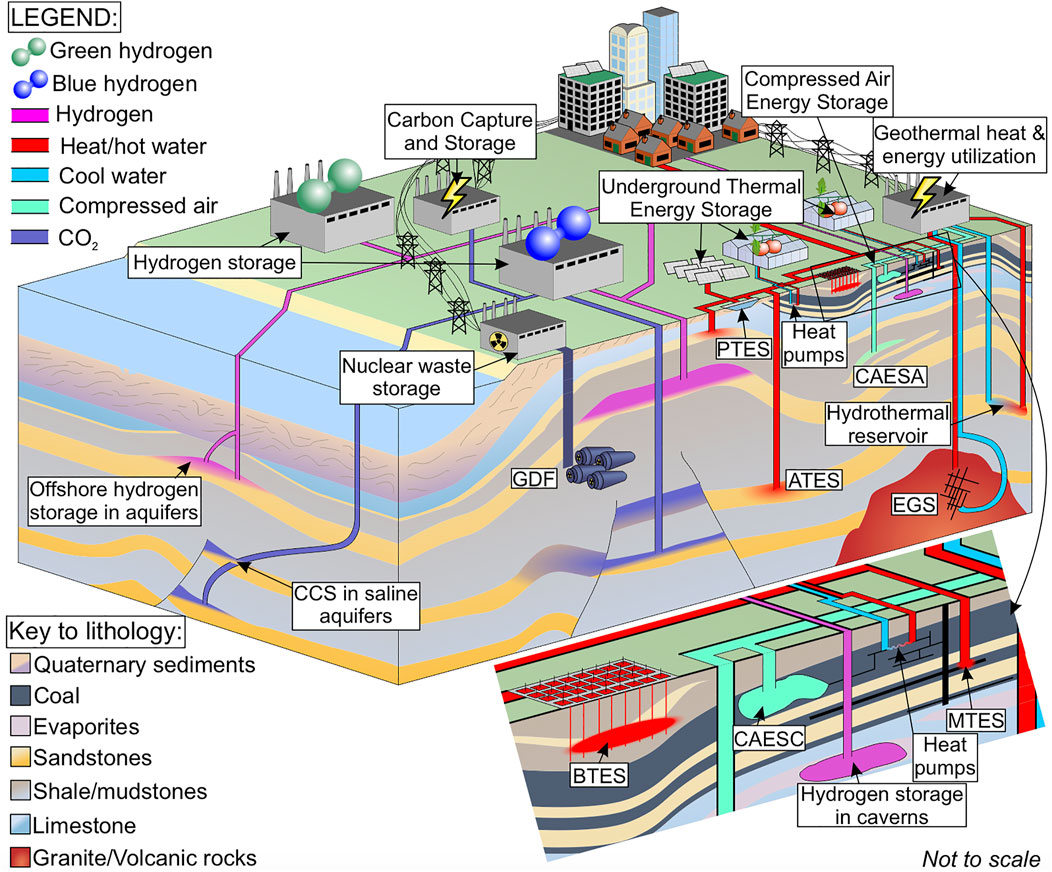
FIGURE 1. Schematic illustrating subsurface utilization to achieve decarbonisation goals for net-zero carbon dioxide emissions. Decarbonisation applications include nuclear waste storage (GDF-Geological Disposal Facility); Carbon Capture and Storage (CCS); geothermal heat and energy utilization (deep Enhanced/Engineered Geothermal Systems (EGS), hydrothermal systems, and shallow (<120 m) geothermal heat pumps); Underground Thermal Energy Storage (ATES, Aquifer Thermal Energy Storage; PTES, Pit Thermal Energy Storage; BTES, Borehole Thermal Energy Storage; MTES, Mine Thermal Energy Storage); Hydrogen storage in caverns and aquifers; and Compressed Air Energy Storage in Caverns (CAESC) and Aquifers (CAESA).
While this review aims to provide an overview of the different physiochemical processes important to consider for the implementation, sustainability and safety of decarbonisation technologies, focus is placed on the strategies to improve our scientific understanding of: 1) how fluids and gases flow and react within a subsurface site as they pertain to selected decarbonisation technologies, and 2) how this flow changes the mechanical properties of the storage site and with that its structural integrity. The field of decarbonizing technologies supported by the subsurface is so wide that it is not feasible to review all possible technologies to the necessary depth. Consequently, we selected three main areas to focus on: 1) geothermal heat and energy extraction including UTES, 2) CO2 storage, and 3) nuclear waste disposal as they cover different types of resource types namely water/steam, gas and nuclear waste, respectively. In addition, the chosen technologies are representative for the range of imposed effects to the environment (e.g., changes in stress field, temperature and geochemistry, air-water-rock reactions etc.), which are shared with many other decarbonisation technologies (Figure 1). Therefore, the concepts, challenges and remaining research questions highlighted in this review will be applicable to the many other decarbonisation technologies that utilize the subsurface. For example, potential structural changes within the host or cap rock due to CO2 storage in saline aquifers may have similar impact to Compressed Air Energy Storage in Aquifers (CAESA) (Figure 1). Hydrogen storage also puts similar pressures on a formation rock as CO2 storage and CAES; they all require high pore spaces within the reservoir rock for injection of large volumes of gas. Therefore, CAESC, CO2 and hydrogen storage in caverns will have similar requirements for a reservoir and also pose similar potential environmental problems.
The determination of subsurface conditions along with current and evolving reservoir rock properties is indispensable for exploration and utilization of decarbonisation technologies. Importantly, the dynamic links between individual THMC processes and reservoir/storage rock properties through time are distinct in different geological materials. This contribution therefore sets out to identify the main THMC processes occurring within the six main lithologies considered for decarbonisation technologies: sandstones, carbonates, granites, shales/mudstones/claystones, evaporites and coal. Even though biological processes also play an important part in the dynamic behaviour of subsurface reservoir, we focus on the physiochemical processes and only the main effects of biological processes are mentioned in the text. All of these processes and their short- and long-term effects have to be taken into account when exploring, implementing and utilizing a sustainable decarbonising technology.
This review is structured in the following way: (1) General overview of main decarbonisation technologies, their geological requirements and technology installation effects on the subsurface; (2) processes and changes within the subsurface strata induced by these decarbonising technologies; (3) solutions for mitigating some of the potential problems associated with point (2), especially those related to permeability decrease within the formation rock over time; and (4) lithology-specific processes occurring within the subsurface that should be considered when evaluating specific subsurface conditions for different technologies. We will discuss and compare benefits and challenges related to different lithologies for each selected decarbonisation technology; and lastly provide an overview of some of the knowledge gaps that are important to fill for successful implementation and operation of these technologies. The review identifies the key parameters and properties that need to be considered when evaluating a specific reservoir rock for the decarbonising technology providing a pathway for research underpinning successful geoscience supported decarbonisation efforts.
Decarbonising Technologies: General Concepts and General Subsurface Requirements
Different technologies require different subsurface characteristics for their successful implementation and operation. Installation and utilization of each technology will create different perturbations to the natural state. The following section describes the general characteristics of each selected decarbonising technology, necessary attributes of the subsurface as well as the imposed effects of these technologies on the strata. For more in-depth reviews on the technologies the readers are referred to additional literature (e.g., Aminu et al., 2017; Apted and Ahn, 2017; Moya et al., 2018).
Geothermal Energy Extraction and Heat Storage
Geothermal heat can be utilized at shallow depth (1.5–300 m) through the use of heat in groundwater and soil via heat pumps, and at greater depth (>500 m) by extracting heat from hot water and/or steam from hydrothermal reservoirs (Figure 1). These may be hosted in sedimentary rocks and typically utilized for direct heat applications or those developed in crystalline rocks, which may also be used to generate electricity as well as direct heat applications. Those in higher temperature (>120°C) but low permeability rocks such as metamorphic or plutonic basement complexes or at the fringe of volcanic hosted geothermal fields are also known as the Enhanced/Engineered Geothermal Systems (EGS) or petrothermal systems, because enhanced fracture networks are created artificially. Pre-existing natural fractures cause induced hydraulic fractures to have multistrands (e.g., Warpinski and Teufel, 1987), and recent core-through experiments show that even in the absence of pre-existing open fractures, stimulation fractures can develop quite complicated patterns, for instance, by bifurcations at bedding planes (Gale et al., 2018). Technologies are available to extract heat from both high and extremely low permeability reservoirs. Geothermal systems are run either by a convection or conduction heat transfer mechanisms (e.g., Moeck, 2014).
Convection-dominated geothermal heat extraction is often associated with a high geothermal gradient (>30°C/km) and natural fluid flow. High rock permeability (>10 mD) is necessary to allow significant convection, which is largely controlled by deformation structures, such as faults and fractures. Such a regime is available at plate tectonic margins, or settings of active tectonism and volcanism (Moeck, 2014, and references therein). These reservoirs have sufficient permeability to transport heat through the convection process because water can flow through the fracture/matrix pore space and heat up through the interaction with the hot rock surface, carrying the then heated water to the boreholes. The drawdown of the groundwater during utilization may significantly limit the amount of heat that can be extracted. Sustainable application of these resources therefore requires reinjection of the fluid after heat has been extracted. Increasing well performance and ensuring the reservoir does not clog up through porosity closure due to suspensions, biofilms or mineral precipitates during production are the main challenges for reservoir engineering in which water flows through the primary porosity within the matrix (e.g., Brehme et al., 2018). In tight systems, permeability is increased either by hydraulic fracturing or acidification (e.g., Zimmermann et al., 2009; Breede et al., 2013; Schumacher and Schulz, 2013). However, it is a challenge of geoscience to be able to document and characterize the pre-existing fracture patterns, so that the optimal stimulation design can be used. Mechanics is a useful tool for deciphering how fracture patterns form. However, fracturing is a physiochemical process that involves the breaking of bonds, and for fractures formed at depths of ∼1–10 km and temperatures of 50–200°C, chemical reactions are common and diverse, making their impact on fracture pattern development hard to predict (Laubach et al., 2019).
Conduction-dominated geothermal heat and energy extraction systems may also be called passive geothermal energy extraction systems due to the absence of fast convective flow of fluids (Moeck, 2014). These systems are normally located at passive tectonic plate settings, where no significant recent tectonism or volcanism occurs. In low porosity reservoirs heat is transferred through heat conduction through rock’s matrix, and sometimes in high porosity reservoirs—through the formation water within the pore system. The low porosity reservoirs are used without the artificially created fracture porosity and rather rely on conduction of heat into boreholes. In this case, permeability of the rock is irrelevant, whereas its conductivity is very important. In such systems, the underground water is static and exchanges heat with a piping loop filled with working fluid via a conduction mechanism (Lund et al., 2004). The buried pipe system acts as a radiator or heat exchanger. At present, a potentially promising technology for closed-loop circulation at great depths is Eavor-Loop™, which consists of two connecting vertical wells several kilometres deep with many horizontal multilateral wellbores several kilometres long (Amaya et al., 2020).
Compared to closed-loop (conduction-dominated) geothermal reservoirs, open-loop (convection-dominated) resources have a higher efficiency of thermal exchange in the subsurface as the heat carrier media is in direct contact with the surrounding ground (Luo, 2014), whereas closed-loop systems only exchange heat at the rock-borehole interface. Therefore, open-loop systems have more impact on the reservoir’s thermo-hydro- mechanical-chemical state, whereas in closed-loop systems, apart from reducing its temperature (e.g., Law et al., 2015), installation and operation of the closed-loop system has a minimal effect on the subsurface strata. Therefore, later in the text we only refer to open-loop systems.
Subsurface Heat Storage—Underground Thermal Energy Storage
Underground Thermal Energy Storage (UTES) uses similar principles and technologies to geothermal energy extraction, except it stores surplus heat energy from power plants and industrial processes, or from installed solar plants or greenhouses instead of utilizing high naturally occurring geothermal gradients. However, in some cases, a hybrid system can use both—geothermal water utilization and heat storage (e.g., Menéndez et al., 2019). The type of UTES depends heavily on the nature of the subsurface storage site, which can be Aquifer Thermal Energy Storage (ATES), Borehole Thermal Energy Storage (BTES), Pit Thermal Energy Storage (PTES), Mine Thermal Energy Storage (MTES) and Cavern Thermal Energy Storage (CTES) systems (Figure 1). ATES uses natural groundwater basins, normally with low natural fluid flow rates, otherwise heat loss would be large (Kallesøe and Vangkilde-Pedersen, 2019). Due to the similarity in flow character, ATES technologies may face the same problems as open-loop geothermal systems. BTES is a closed-loop system and its principle is to heat or cool the subsurface by circulating a fluid in plastic U-tube pipes installed in a large number of closely spaced boreholes (e.g., Rad et al., 2017). The system uses the subsurface itself as the storage material, which can range from unconsolidated sediments to rock with or without groundwater. In this type of storage medium, the heat transport mechanism is heat conduction and thus the problems associated with BTES installation are similar to the ones occurring within closed-loop geothermal systems. PTES works by storing hot water in very large excavated basins with an insulated lid. The sides and bottom are typically insulated by a polymer liner or concrete (e.g., Sørensen and Schmidt, 2018). PTES is less dependent on the subsurface characteristics themselves except for the requirement of low thermal conductivity. However, their shallow position in the subsurface may lead to communication with groundwater flow which will result in significant stored heat losses (Kallesøe and Vangkilde-Pedersen, 2019). MTES is similar to PTES, but the system utilizes abandoned mines. CTES is used for heat storage systems that utilize any underground “cavities,” may it be natural such as karstic formations or man-made, e.g., insulated tanks buried underground. For CTES to be energy efficient cavities must not be connected to subsurface water systems.
Carbon Capture and Storage
Since the 1970’s, CO2 has been injected into geological formations for enhanced oil recovery (EOR), typically after water flooding as a tertiary recovery mechanism (e.g., Hill et al., 2013). The injected CO2 decreases oil viscosity and density, resulting in improved fluidity and enhanced lifting and extraction of oil. Despite the long use of CO2 for EOR, the long-term storage of CO2 is a relatively new concept, with the first CO2 storage facility, Sleipner Vest, starting in 1996 (Korbøl and Kaddour, 1995). Storage of CO2 is considered an essential step in limiting global warming to 1.5°C, with three out of four scenarios to net-zero emissions involving CO2 sequestration (IPCC, Forthcoming 2018).
Formations suitable for CO2 storage must be porous and permeable to allow injection of large volumes of CO2 (>1 Mt of CO2), and must have effective trapping mechanisms in place preventing leakage to the atmosphere (Hepple and Benson, 2005) or adjacent groundwater (Ardelan and Steinnes, 2010; Lu et al., 2012; Trautz et al., 2013). Several trapping mechanisms may retain CO2 (Figure 2): (a) stratigraphic and structural traps (by buoyancy effect); (b) residual (trapped in rock pores by water capillary pressure); (c) solubility (residual gas trapping by dissolution), and (d) mineralization (changing the pore-space topology and connectivity) (e.g., Burnside and Naylor, 2014; Sharma et al., 2017). These trapping processes take place at different rates, and provide increasing storage security. However, they show a decreasing order of overall contribution to CO2 trapping (i.e., structural trapping being the most important volume-wise, mineral trapping making the least contribution). Mineral dissolution-precipitation reaction rates are relatively slow thus mineral trapping would only become important at a geological timescale (Zhang and Song, 2014). In addition, the presence of high concentrations of natural CO2 in subsurface reservoirs that have no evidence of enhanced diagenesis (e.g., Wilkinson et al., 2009) suggests that in many, if not most, case mineralization is not an effective storage mechanism.
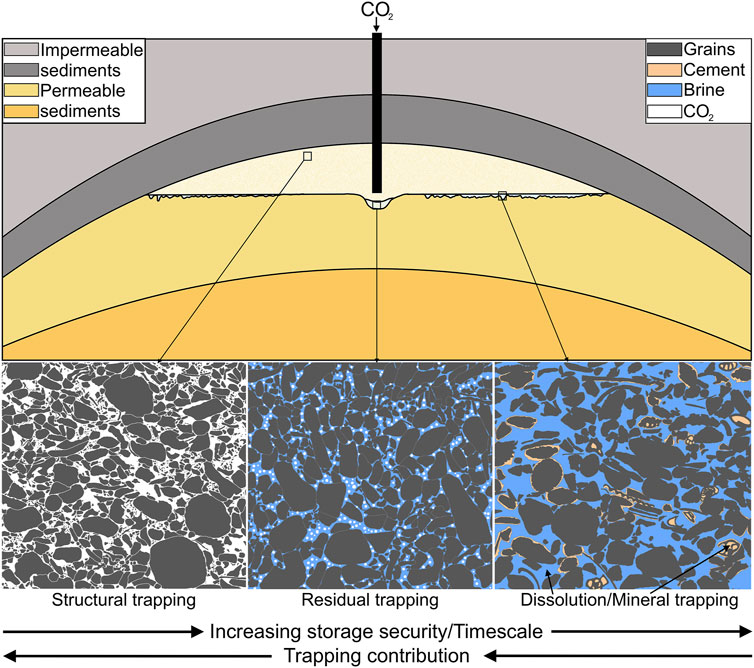
FIGURE 2. CO2 trapping mechanisms: structural, residual, dissolution and mineral trapping. These mechanisms show an increasing order of storage security and timescales it takes for the CO2 to be trapped by that mechanism (i.e., CO2 gets trapped by stratigraphic and structural traps on a field operation timescales, whereas mineral trapping only becomes important at a geological timescale). Note, that these mechanisms, however, show a decreasing order of overall contribution to CO2 trapping.
Storage in abandoned oil and gas fields is particularly attractive because they are well characterized and the fact that they have retained hydrocarbons is strong evidence that they have good seals. It is also the case that depleted gas reservoirs have larger pore volumes available for storage compared to, for instance, saline aquifers of the same size whose porosity is completely filled with water. Although having retained hydrocarbons over geological-time is not a guarantee that CO2 will not leak because: 1) in the near vicinity of wells the sealing capacity of the cap rock may have been damaged by the penetration of wells (Metcalfe et al., 2017); 2) CO2/CH4/brine interfacial tension is much lower than hydrocarbon-water systems (Li et al., 2006), 3) CO2 may have a different wettability to hydrocarbons being less water-wet; 4) reactions at the shale interface may compromise seal integrity (e.g., Gholami et al., 2021) and 5) the reduced horizontal stresses within the depleted reservoirs add difficulty in drilling and cementing increasing the risk of well leakage that may also lead to large mud losses and difficulties in cemented casing (e.g., Shahbazi et al., 2020).
Injected CO2 is cold: CO2 turns from a gas into a liquid by compressing it to the corresponding liquefaction pressure at temperatures between −56.5°C and 31.1°C (e.g., Balat and Öz, 2007). Therefore, during CO2 injection the pressure near the injection well declines rapidly as gas expands into the reservoir, resulting in lower temperature of the reservoir (Vilarrasa and Rutqvist, 2017). The long-term effects of the Joule-Thomson cooling on the reservoir are still poorly understood, however it is clear that it will change the physical properties of the rock around the injection well and within the reservoir (e.g., Chen et al., 2020). For instance, induced thermal contraction of the reservoir matrix could lead to the opening of pre-existing, or formation of new fractures, potentially forming leakage pathways (e.g., Vilarrasa et al., 2017; Salimzadeh et al., 2018), negating the assumption that leakage is not at risk from depleted oil and gas reservoirs (Lokhorst and Wildenborg, 2005). As long as the reservoir pressure is below the hydrostatic pressure, leakage will occur by diffusion only (e.g., Busch et al., 2010).
Combined Technologies: Geothermal Energy and Carbon Capture and Storage
Injecting geothermal waste fluids together with high amounts of dissolved CO2 may often prove effective for the underground disposal of CO2, as well as for maintaining field pressures (Wu and Li, 2020). CO2 on this own in a supercritical condition (scCO2) has also been proposed as a working fluid in the geothermal systems (Pruess, 2006). The advantage of using scCO2 as opposed to water is its lower viscosity, larger compressibility and expansivity, which would increase buoyancy forces and reduce the parasitic power consumption of the fluid circulation system, as well as the reduced reactivity with rock (Lo Re ́et al., 2014). Unlike water, CO2 is not an ionic solvent, thus it is predicted to reduce the potential for dissolution and subsequent re-precipitation of minerals, avoiding problems of scaling and formation plugging (Brown, 2000). However, using scCO2 instead of water would increase compression costs associated with re-injection.
Nuclear Waste Disposal
European Commission signed a Taxonomy Complementary Climate Delegated Act in February 2022, stating that nuclear power will be added to taxonomy under certain requirements one being a secure long-term radioactive waste disposal. Therefore, finding ways to safely store nuclear waste in geological formations is becoming ever more important. Geological repositories for nuclear waste disposal need to fulfil a number of criteria, including low permeability of the host rock, protection against groundwater contamination, and long-term mechanical, physical and chemical stability (Borojević Šostarić and Neubauer, 2012). If these requirements are not met, and there is a contact between the groundwater and nuclear waste, radionuclides may be released into the environment, threatening life. However, even a contact of water with the containers is potentially dangerous because hydrogen gas generated by the corrosion may release gaseous C-14 (Schwartz, 2012) or result in the formation of fractures that could compromise the integrity of the site. Detailed site investigations must include descriptive models of the initial state of variables such as geology, hydrogeology, hydro-geochemical features, and mechanical and thermal gas transportation factors (Ewing et al., 2016). Rock types having suitable properties for the long-term Geological Disposal Facility (GDF) are evaporites, shales and crystalline basement rocks (Figure 1; Borojević Šostarić and Neubauer, 2012). The depth of most GDF’s is expected to be around 200–900 m (e.g., Sellin and Leupin, 2013; NDA, 2016a; Scourfield et al., 2020). Containers holding radioactive waste may comprise synrock (synthetic rock), stainless steel, copper, etc. (e.g., Ragheb, 2011). At closure, disposal containers may be placed either in relatively dry conditions, such as in an evaporite host rock, where a backfill material is typically used to seal the container, or in contact with wet buffer materials, e.g., cement or bentonite (e.g., NDA, 2016a; Groff et al., 2016). Bentonite is a clay which swells at saturated conditions. The bentonite buffer may swell to the point where it completely seals the space between the waste container and the host rock (NDA, 2016b). If the bentonite buffer forms a seal barrier, it may inhibit microbiological activity around the disposal container, thus protecting it from corrosion by limiting the flux of corrosive species, such as sulphides, to the container surface. The function of the buffer material is to adsorb any nuclides and close potential fractures, and buffer nuclear waste chemically, reducing the reactivity of groundwater that may come into contact with the containers. After sealing of vaults and access tunnels and closure of the GDF, dissolved oxygen will be present in the GDF system due to air intrusion into the system (Smith, 2015). Extensive use of cement in a GDF, potentially as a backfill material around waste packages and in access tunnels and other structures, may increase pH of groundwater due to saturation of the GDF, which may lead to the formation of a hyperalkaline plume with a pH of up to 13.5 (van Aardt and Visser, 1977; Savage et al., 2002). Increased alkalinity may alter the radionuclide retention capacity of the rock, because radionuclides are soluble in higher pH environments (Smith, 2015). The pH around a GDF would stabilise after a few thousand years following mineralisation. Therefore, the surrounding backfill material of the GDF must be designed in accordance to precise models of expected changes to the surrounding geology (Ewing et al., 2016).
Heat generated from the nuclear waste package elevates temperatures within the container, nearby engineered structures and the surrounding host formation; temperatures are predicted to reach up to 180–200°C (NDA, 2016a). The temperatures in the near field strongly depend upon the properties of the material used, such as waste form, container and backfill material, and the host rock properties (Okamoto et al., 1991). Thermal parameters of rock mainly depend on its mineral composition, microstructure and porosity, which is closely related to the loss of water and structural damage caused by thermal reactions, such as evaporation, material phase change and chemical reactions (Sun et al., 2016). Evaporites, granite and clay-rich shale show thermal conductivity in a decreasing order (Okamoto et al., 1991), governing the temperature gradient surrounding the repository.
Geoscience plays a central role in evaluating the GDF (e.g., predicting the behaviour of groundwater systems in glacial periods), and in modelling the near-field response of the surrounding host rock of a GDF including the excavation damage zones, effect of heat flux and the extent of rock desaturation during the GDF operational period (Stephenson et al., 2019). A model for the evolution of fluid chemistry and mineral alteration in the environment around the GDF should consider the flow of water, gas and heat, reactions between minerals, CO2 gas and aqueous species, as well as porosity-permeability-capillary pressure coupling for a dual permeability (fractures and matrix) medium (Spycher et al., 2003). Risk assessment of disposing nuclear waste involves a detailed study of geological processes occurring now and in the recent past to understand changes up to 1 myr into the future as required by the regulators. Degree of seismic activity has to be assessed through time, as well as effects of glaciation, uplift and erosion. Climate change will influence sea-level rise so it should also be considered.
Processes and Changes Within the Subsurface Strata Induced by Decarbonising Technologies
Geological strata are defined by physical, chemical, mechanical and biological boundary conditions that have generally operated over very long timescales (i.e., millions of years). Field operations change the subsurface far more rapidly. Small changes in the THMC state may have a significant impact on fluid flow and hence sustainability of the decarbonisation system. Installation of each technology has a different effect on the subsurface and will create different perturbations to the natural state. The following section describes the effect of different processes induced by the installation of the selected decarbonising technologies on subsurface characteristics and technology sustainability. Key problems and processes that need to be taken into account when installing these technologies are explained in more detail below and summarised in Table 1.
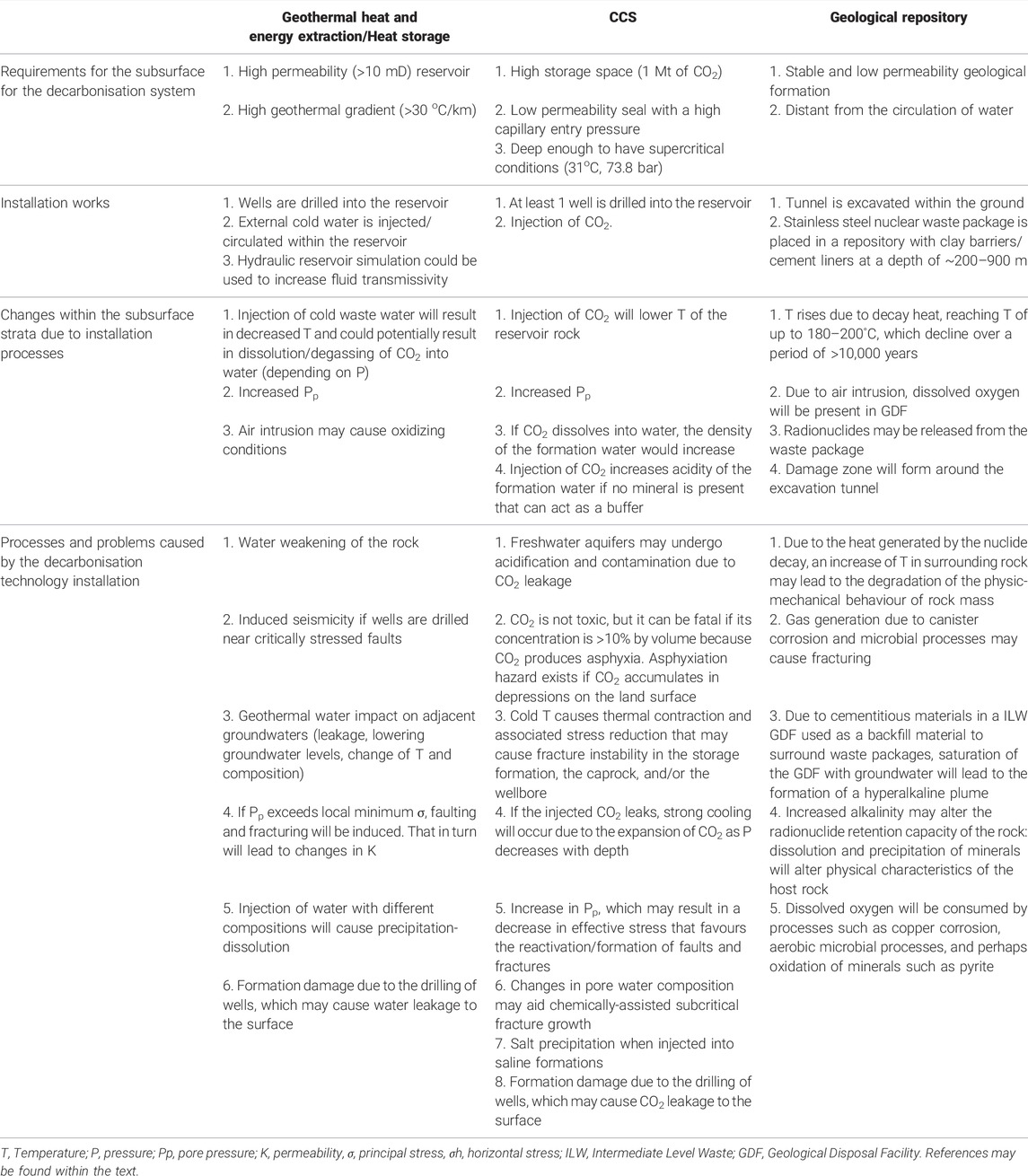
TABLE 1. Summary of the subsurface requirements when installing a decarbonisation technology (geothermal heat and energy extraction, heat storage, CCS and nuclear waste disposal), technology installation works, changes within the subsurface strata caused by these works, and processes and problems that follow the installation.
Geothermal Heat and Energy Extraction and Heat Storage Systems
Dissolution-Precipitation in Pore Space
In open-loop systems, changes in chemical composition and temperature of the reinjection water may induce a series of interactions between the reservoir rock, residing fluids and the reinjected water due to chemical local disequilibrium, which may impact the porosity and permeability of a reservoir (e.g., Grigsby et al., 1983; McCartney, 1987; Su et al., 2018; Brehme et al., 2018; Brehme et al., 2019). After water is injected into the reservoir, the rock and the fluid system will attempt to re-establish equilibrium conditions by dissolution of minerals in the host rock, and/or formation of secondary minerals. The mineralogy of the rock will determine the exact nature of the reactions that will occur. Some minerals become more supersaturated on cooling and hence are at risk of precipitating during fluid convection: prominent amongst these are the sulphate minerals, silica, barite, and gypsum (e.g., Burton and Walter, 1987; Arnórsson, 1989; Brehme et al., 2019; Setiawan et al., 2019). Calcium and sulphate are both common solutes in water so gypsum may be the prime suspect for chemical scaling on cooling. Calcite is one of the most chemically reactive minerals, thus calcite reactions are the most common in carbonate-rich reservoirs (e.g., Liu and Zhao, 2000). In an open system, calcite solubility is retrograde, meaning that calcite solubility increases with decreasing temperature (Wood, 1986). Consequently, cold water injection may result in dissolution of calcite. Taron and Elsworth, (2013) suggested that in the EGS systems thermal-hydro-mechanical fracture dilation dominates reservoir performance at the beginning of its exploitation, whereas chemical precipitation in the vicinity of the wellbore may affect the long-term reservoir performance.
Redox Reactions at Fluid-Rock Interfaces
Air introduction into reservoirs due to poor sealing of reinjection wells or water delivery pipelines may lead to increase in dissolved oxygen and initiation of redox reactions. Contact between the oxygen and dissolved reduced metals in the geothermal systems risks the precipitation of manganese oxides (MnO2) or ferric oxyhydroxides (Fe(OH)3), and the risk of biofilm formation (Brehme et al., 2019). Fe dissolution and subsequent precipitation of minerals such as goethite or siderite result in changes in ionic composition and pH of the water, as well as heating of the water if the reaction is exothermic (e.g., Su et al., 2018). Therefore, air intrusion into geothermal reservoirs may result in an increased acidity and scaling of iron-bearing phases (e.g., Banks et al., 1997).
Decrease of Mechanical Strength and Stiffness
Injection of external water into a formation rock will change mechanical state of the reservoir. Laboratory rock mechanics tests demonstrate that water commonly weakens rocks, including reducing strength and stiffness (e.g., Wong et al., 2016), enhancing creep and plastic deformation, and accelerating failure rates even at low temperatures (<200°C) (Brantut et al., 2013). This water-weakening behaviour is attributed to water facilitating subcritical fracture growth, or activating fluid-assisted deformation through stress corrosion (Wiederhorn, 1967), dissolution (Simmons and Freiman, 1981), and/or microplasticity (Schubnel et al., 2005). Therefore, water injection into, for instance, an abandoned gas reservoir may greatly reduce its yield stress, resulting in wellbore collapse, and fracturing and faulting of the reservoir rock potentially coupled with induced seismicity (e.g., Majer et al., 2007; Kwiatek et al., 2019; see more details in Induced Seismicity section).
Pore Pressure Increase Causing Fracturing and Faulting
During injection of external fluid, the reservoir stress path may be affected by the minimum horizontal total stress changes resulting from pore pressure fluctuations and by the change in total vertical stress during expansion of the reservoir resulting from stress arching. The changes in deviatoric stresses may lead to faulting and fracturing (e.g., De Simone et al., 2013). Such failure may result in increased or decreased reservoir permeability, reactivation of existing faults and fractures which may breach the hydraulic integrity of the caprocks that bound the reservoir (Soltanzadeh and Hawkes, 2008) and induce seismicity (see Induced Seismicity section).
Temperature Effects on Reservoir Stability
The injection of cold water into a hot reservoir induces thermal stresses due to rock contraction. An area of contraction increases with time following thermal diffusion (Parisio et al., 2019). Thermal effects induce a significant perturbation on the stress field, creating local fractures that can increase injectivity index (Pasikki and Pasaribu, 2014), or even trigger induced seismicity in the surroundings of critically oriented faults near the injection well (De Simone et al., 2013).
Hydrological Effects on Adjacent Groundwaters
Open-loop geothermal and UTES systems may cause a range of environmental changes to the adjacent groundwater systems. Long-term abstraction where extraction is larger than injection may lower regional groundwater levels and have an impact on local drinking water wells (Preene and Younger, 2014). Communication of a geothermal or heat store reservoir with a regional groundwater system may cause pollution problems due to precipitation of dissolved chemicals or release of dissolved gases related to external fluid injection. Open-loop systems may also affect aquatic ecology in the groundwaters due to chemical variations such as increased oxygen content (Preene and Younger, 2014). Moreover, injection of external water may cause long-term changes in groundwater temperature. This in turn may cause geochemical perturbations due to changes in chemical equilibria between the groundwater and reservoir rock, which may affect water quality in the aquifer.
Induced Seismicity
Currently the biggest public concern associated with EGS technologies is a possibility of induced seismicity. It should be noted that the term induced seismicity is currently very broadly applied and includes seismicity that can be recorded at the Earths’ surface. The instrumentation used is highly sensitive and records seismicity from day-to-day traffic and tidal modulations (e.g., Lecocq et al., 2020). By far, most of the recorded induced seismicity is observed in projects circulating fluid through basement rocks or carbonates (e.g., Basel, Soultz-sous-Forêts, Landau, Insheim, Rittershoffen), whereas circulation through the matrix of sedimentary rocks tends to be less seismogenic (e.g., Evans et al., 2012; Baisch et al., 2016; Diehl et al., 2017). However, in most cases, induced seismicity will not exceed a local magnitude, ML, of 3.0, with only a handful of events with ML>3.0, the strongest one striking the city of Pohang in 2017 with ML = 5.5 (e.g., Grigoli et al., 2018; Buijze et al., 2019; Parisio et al., 2019). High impact seismic activity, including seismic activity inducing infrastructure damage, tend to occur on pre-existing local fault systems (e.g., Evans et al., 2012). This is because less strain energy is required to trigger slip on a pre-existing fault surface than to create a new fracture within an intact geological unit. Geothermal wells drilled near critically stressed faults, in particular larger scale faults (e.g., lateral fault extension >1 km), may thus cause high magnitude earthquakes (Baisch et al., 2016). The authors note that in absence of critically stressed faults, even large volume fluid injections do not induce any measurable seismicity. Some geothermal energy projects, such as the United South Downs project in Cornwall, UK, specifically target faults due to their high permeability (e.g., Richards et al., 1992). Identifying critically stressed faults has practical limitations: even a 3D seismic survey will not necessarily detect all faults of a size that is relevant for the seismic hazard and most faults resolved in a seismic survey will not be critically stressed (Baisch et al., 2016). Moreover, assessing the fault strength (i.e., coefficient of friction and cohesion) is difficult using existing geophysical exploration technologies.
Carbon Capture and Storage and Combined Carbon Capture and Storage/Geothermal System
CO2 Leakage From Injected Reservoir to Surroundings
Although CO2 is not toxic it can be fatal if its concentration exceeds 10% by volume, as it causes asphyxia (Baxter et al., 1999). A leakage could cause CO2 to accumulate in topographic depressions on the Earths’ surface as it is heavier than air, which can cause adverse ecological effects such as damaging plant and soil microbiology (Roberts and Stalker, 2017). Freshwater aquifers may undergo acidification and contamination due to CO2 leakage. If the injected CO2 leaks through faults reaching lower confined pressure, strong cooling will occur due to the expansion of CO2 as pressure decreases with depth (Vilarrasa and Rutqvist, 2017), resulting in a number of problems (see Decreased Temperature section).
Decreased Temperature
Cold CO2 injection induces thermal contraction and associated stress reduction that may cause fracture instability in the storage formation, the caprock, and/or the wellbore (Vilarrasa and Rutqvist, 2017). If thermal cycling occurs as a result of alternating periods of CO2 injection with shut-downs, causing heating and cooling, radial fractures or debonding of the cement may occur, potentially leading to CO2 leakage (Vilarrasa and Rutqvist, 2017).
Increased Pore Pressure and Regional Fluid Flow
Reservoirs may be divided into those with regional fluid flow and pressure connectivity (Green et al., 2016; Heinemann et al., 2016), and isolated reservoirs with limited regional connectivity (Swarbrick et al., 2005, 2010). Isolated reservoirs have a limited storage capacity, as injecting too much CO2 could increase pore pressure potentially leading to top seal failure through hydraulic fracturing, or fault reactivation (Swarbrick et al., 2013). With careful pressure management, isolated reservoirs could make for good long-term storage, as long as the top and lateral seals have sufficiently low relative permeability to CO2-water mixture fluids (Swarbrick et al., 2013; Karolytė et al., 2020). Reservoirs with regional pressure connectivity do pose some risk for long-term CO2 storage because they are susceptible to reservoir hydrodynamic flow, which may tilt fluid contacts beyond spill points, leading to reservoir leakage (Green et al., 2016; Heinemann et al., 2016). Care must be taken on injecting CO2 into a hydrodynamic reservoir as the increase in pressure due to injection may alter hydrodynamic flows in another part of the reservoir, especially within narrow, contained reservoirs such as the Captain Sandstone (Williams et al., 2016).
CO2 Induced Acidification
Injection of CO2 increases acidity of the formation water through the following reaction: CO2 + H2O = H2CO3 (Gunter et al., 2004). CO2-rich fluids may be highly chemically reactive in particular lithologies, impacting reservoir permeability and porosity by dissolution and precipitation reactions. For example, carbonate minerals are the fastest minerals to respond to the changes induced by CO2 injection (Gunter et al., 2004). These minerals, if present, may dissolve and thus buffer acidity (Banks et al., 1997). Changes in pore water composition associated with CO2 injection may also aid chemically-assisted subcritical fracture growth (Chen et al., 2020).
Salt Precipitation
Salt may precipitate around the injection well when CO2 is injected into deep saline formations (Vilarrasa and Rutqvist, 2017). If water is present in the system, the water will tend to evaporate into the dry CO2, increasing the NaCl concentration in the liquid phase. Once the equilibrium solubility is reached, salt may precipitate closing the pore throats and hence resulting in a decreased porosity and permeability in particular (Vilarrasa and Rutqvist, 2017).
Induced Seismicity
The relationship between long-term injection and induced seismicity has been documented (Kaven et al., 2015; Taylor et al., 2018), suggesting an increased probability of earthquakes triggered by large injections of CO2 into the brittle rocks found in continental interiors, threatening the seal integrity (Zoback and Gorelick, 2012). However, Vilarrasa and Carrera, (2015) argue that large earthquakes due to geologic CO2 storage are unlikely because (i) soft sedimentary formations are rarely critically stressed; (ii) the most unstable conditions occur at the beginning of injection thus it can be controlled; (iii) CO2 dissolution into brine may help in reducing overpressure; and (iv) CO2 will not flow across the cap rock due to capillary pressure, but brine will, which will reduce overpressure further. Therefore, overpressures caused by CO2 injection will most likely dissipate with time, making the induced seismicity an unlikely scenario (Vilarrasa and Carrera, 2015).
Nuclear Waste Disposal System
Increased Temperature
High temperatures generated by nuclide decay will be retained in the engineered barrier system for long periods of time (>10,000 years) (NDA, 2016a), leading to temperature gradients and localized dehydration processes, which will tend to dry the rock, cause degradation of the physio-mechanical behaviour of rock mass, create local pore overpressure, and change the natural permeability of the host-rock (e.g., Tsang et al., 2012). Heating of the rock causes its expansion, which pushes out the cooler surrounding rock, resulting in induced tension in the cooler rock and additional compressive stresses in the hotter rock (Okamoto et al., 1991). These effects may be beneficial in that expansion will close cracks in the hotter region, reducing the fluid flow to and from the waste in the GDF. However, the tensions will open the existing fractures between the ground surface and the GDF, resulting in increased permeability in the far field of the rock cover. The thermomechanical response of the overburden rock could also result in the opening of existing fractures near the surface due to nonlinear uplift. The resultant increase in near surface fracture permeability may affect the water-flow patterns, perturb any microbial populations contained within the original undisturbed system and those introduced during construction of the repository (Okamoto et al., 1991). Upon heating and boiling, CO2 exsolution from pore waters may raise pH and cause calcite precipitation (Spycher et al., 2003). Heat may also enhance dissolution of silica minerals, and increase smectite to illite conversion rate (e.g., Huang et al., 1993).
Increased Alkalinity
Cement used for backfilling the nuclear waste package will increase pH of the surrounding formation waters in the GDF, forming an alkaline disturbed zone (Bateman et al., 1999). If the hyperalkaline pore fluid encounters unaltered groundwater, dissolution of primary minerals such as K-feldspar will occur, together with the precipitation of secondary phases such as carbonate or gypsum (Savage and Rochelle, 1993; Techer et al., 2006). That will lead to alteration of porosity and permeability of the host rock, and potentially alter the radionuclide retention capacity of the rock (Montori et al., 2008). Moreover, high pH pore water interactions with bentonite clay may facilitate the formation of colloids, potentially increasing the movement of radionuclides in a repository environment (Missana et al., 2011).
Redox Reactions
Some oxygen is likely to be entrapped in the GDF construction and waste materials due to air intrusion into the system, leading to redox conditions initially similar to those of naturally aerated systems. Dissolved oxygen will be consumed by the processes such as corrosion of copper containers, aerobic microbial processes, and oxidation of minerals such as pyrite (Yang et al., 2007; Hall et al., 2021). Estimates of the maximum amount of oxygen potentially trapped in the GDF after closure can be used to evaluate the maximum amount of corrosion expected on waste containers during this early post-closure period (NDA, 2016a). Values of the order of 1–10 mol per m2 surface area of the container, amounting to a maximum depth of corrosion of the order of 10–100 µm if uniformly distributed over the container, were estimated by King, (2007). The hyper-alkaline pore fluids will facilitate degradation of cellulosic materials, providing substrates for microbial metabolism. The microbial-mediated oxidation of organic matter rapidly causes the depletion of oxygen in the system (Duro et al., 2014). Corrosion of wastes and canisters along with microbial processes will begin to generate gases (see Gas Generation section). Over time, conditions in a GDF will eventually become reducing as oxygen will be consumed by redox reactions (Wersin et al., 1994).
Gas Generation
Depending on the nature of the waste materials and the ambient conditions, a number of different gases may be produced from a nuclear waste package due to corrosion of metallic canisters and microbial processes (NDA, 2016b). These gases include hydrogen, carbon dioxide, methane as well as small amounts of hydrogen sulphide and radon (Metcalfe et al., 2008). The generated gas may accumulate and as pressure increases will start flowing through the engineered barrier into the surrounding rock (Gens et al., 2001). It is also possible that gas pressures could increase sufficiently to result in hydraulic fracturing, contributing to the positive feedback loop in the coupled fracture-transport, and hence possible radionuclide release (Olivella and Alonso, 2004).
Radionuclide Leakage
In an event of radionuclide leakage from the GDF, groundwater represents the most effective media through which radionuclides can be transferred to the surrounding environment (e.g., Benbow et al., 2014). The mixing of released radionuclides with groundwater depends on the depth of groundwater table in the area where GDF is located. The problem of radionuclide mixing with the unsaturated moisture content becomes complicated because vadose zone may promote sorption, biodegradation and transformation of radionuclides due to the presence of an elevated organic matter and clay content (e.g., Suresh Kumar, 2015). Therefore, radionuclide transport processes could potentially include advection, hydrodynamic dispersion, sorption, decay, and matrix diffusion. The coupling between geology (microstructural properties of the rock and hydraulically connected fracture system), hydrogeology (flow), and hydrochemistry (reactivity of the solubilities of radionuclides and chemical reactions of nuclides with geological materials) play a crucial role in evaluating mobility and spreading of wastes within the subsurface (e.g., Suresh Kumar, 2015). Such transport processes need to be investigated in detail building upon dual-porosity systems (e.g., Natarajan and Kumar, 2010; Natarajan and Kumar, 2012).
Damage During Excavation
An excavation damage zone may form during the GDF construction (Tsang et al., 2005). This damage zone represents a region of enhanced permeability caused by the formation of tensile or shear fractures (e.g., Bossart et al., 2004; Marschall et al., 2008). An initial increase in permeability of 4–6 orders of magnitude has been measured at Mont Terri (Tsang et al., 2012), Bure underground research laboratories (Armand et al., 2007) and Tournemire (Matray et al., 2007). The extent and intensity of the excavation damage zone depend on several factors, such as mechanical properties and heterogeneity of the host rock, the anisotropy of the stress field, over-consolidation ratio, the presence of bedding planes, and the engineering technique used to excavate (e.g., Popp et al., 2008; Tsang et al., 2012). However, this effect decreases over time because of clay swelling and creep especially in cases where swelling refill materials are used to provide a back pressure on the rock (e.g., Lanyon et al., 2009; Tsang et al., 2012).
Lithology-Specific Processes Associated With Decarbonisation Technology Installations
Each of the processes identified above will show varying effects and dynamics within different lithologies. Therefore, in the following section, we summarize the nature of these key processes and their effect on the mechanical integrity and sustainability of decarbonisation technologies and reservoir hydraulic properties within several rock types most commonly used for the decarbonisation applications discussed in the paper. Decarbonising Technologies: General Concepts and General Subsurface Requirements section (above) shows that the majority of the processes are universal to the different technologies—they all affect fluid flow, temperature and stress changes in the subsurface. Therefore, typical behaviour is described in terms of these main processes, and only when necessary, processes and dynamics are highlighted that are specific to a certain technology.
Sandstones
Sandstones are mainly composed of a sand-size grains of quartz with variable amounts of feldspar, mica and lithic fragments; the spaces between which may be filled with cement of silica, carbonate or clays. Sandstones invariably contain impurities at different scales, where arenites (grain scale) might be mixed with mm to dm layers of clay or impure sandstone. Porosity of a quartzose sandstone can be predicted with some degree of accuracy using parameters such as depth, temperature gradient, burial rate, stylolite frequency (e.g., Bjørkum et al., 1998), composition and texture of the sandstone upon deposition etc. (e.g., Lander and Walderhaug, 1999). Impure sandstones are more difficult to characterise.
The majority of sandstone reservoirs are characterized by the dominance of intergranular porosity, and hence matrix permeability (Ehrenberg and Nadeau, 2005). Sandstone reservoirs may provide suitable hydrothermal systems for geothermal heat and energy extraction and CCS, as sufficient permeability is often already in place (e.g., Nielsen et al., 2004; Feldrappe et al., 2007; Donselaar et al., 2015). Heat in these sandstones is thus largely transferred by conduction through fluid flow through the rock matrix and formation fluids, whereas fractures may add additional high-efficiency pathways (Figure 3A). Unless it is a tight sandstone (porosity <10%, intrinsic permeability <0.1 mD), where faults and fractures play a crucial role in creating efficient permeability because pre-existing pore throats within the matrix range from nano- to micro-scale and forms poorly connected network (e.g., Lai et al., 2018). In this case, the reservoir acts as a EGS/petrothermal system and hydraulic stimulation is necessary to make the reservoir hydraulically efficient.
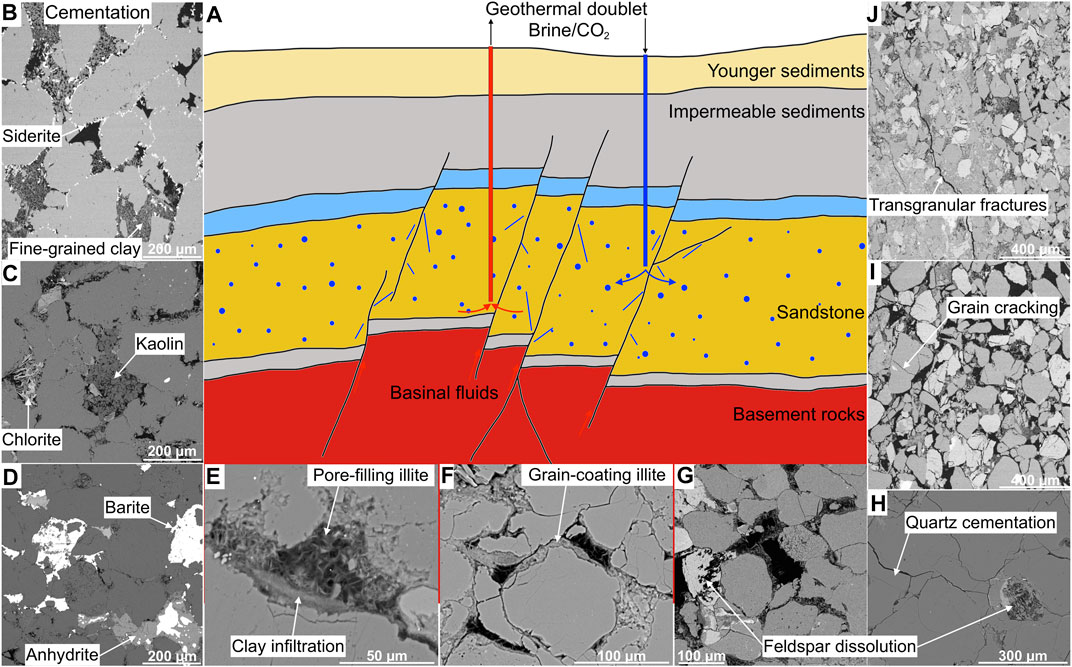
FIGURE 3. (A) Schematic illustration of a geothermal and/or CCS system in a sandstone reservoir, showing structural features and groundwater flow paths. Arrows indicate flow direction, and blue to red colours indicate cold and hot water, respectively. (B–J) SEM images represent examples of products of the THMC processes that may occur within sandstones during the geothermal and/or CCS technology installation/operation: (B–H) Products of mineral dissolution and precipitation; (I,J) Products of mechanical changes within the reservoir.
Water-rock interactions in sandstones, induced by changes in chemical composition and redox conditions of injected water, include precipitation of secondary clay and hydrothermal minerals, such as chalcedony, calcite, gypsum and other minerals with high reaction rates (Figures 3B–H; Dou, 2012; Li et al., 2012; Brehme et al., 2018). Feldspars are the most reactive primary minerals, and their dissolution plays an important role for the generation of quartz cement as well as illitization of clays at a deeper depth with high temperature (Kasztelewicz and Tomaszewska, 2019). Changes in electrostatic forces between mineral surfaces may cause mobilization of clays leading to changes in permeability (Figures 3E,F; e.g., Wilson et al., 2014).
Introduction of air into reservoirs may lead to increase in dissolved oxygen and initiate redox reactions. For instance, the oxidation of pyrite results in the formation of iron oxides and sulphuric acid (Plumlee, 1999). Siderite is fairly stable in oxidizing groundwater because even if it dissolves the local precipitation of Fe oxides tends to create crusts and slow the reaction. Dissolved oxygen and redox reactions will result in changes in ionic composition and pH of the water, which will promote dissolution of carbonates (Plumlee, 1999). Major et al. (2018) studied CO2-water-rock interactions within sandstones at the Crystal Geyser and Salt Wash field sites in the USA, where geothermal water haven been flowing over long timescales (>102–104 yrs). The authors observed hematite cement dissolution and preferred bleaching in the vicinity of CO2 springs flowing along fault zones. Dissolution of iron-bearing phases has also been inferred by the geochemistry of produced water in the Rangely Field, Colorado, after several months of CO2 flooding (Bowker and Shuler, 1991). Despite dissolution of Fe-oxide minerals, geothermal springs may also cause precipitation of calcite (Baer and Rigby, 1978; Urquhart, 2011), destruction of chlorite in the lithic fragments and net corrosion of feldspars, as well as an increase in the concentration of siderite and ankerite (Figures 3B–H; e.g., Watson et al., 2004).
In hot springs close to volcanic areas, silica-rich fluids can flow along fault zones and cause hydrothermal silicification (Guido and Campbell, 2018). Here magmatic activity supplies with a high temperature required to dissolve the silica and favour the kinetics of the chemical reactions necessary for the release of silica in sufficient quantities to affect the lithologies crosscut by the fault zones. Menezes et al. (2019) studied such hydrothermal silicification along the Afonso Bezerra strike-slip fault system in the Potiguar Basin, Brazil, to assess the role of Si-rich fluids in fault geometry, properties, and evolution. The authors observed that in faulted sandstones an intense quartz cementation process occurs by replacing the matrix with polymicrocrystalline silica and by grain overgrowth (e.g., Figure 3H). Menezes et al. (2019) compared the mineralogy of the host rock and the rock affected by the fault, and found absence of the plagioclase and the microcline in silicified sandstones and the appearance of clay minerals, such as illite. Pervasive silicification and cementation of quartz, opal, and chalcedony cause the destruction of porosity and permeability, making the fault zone behave as a barrier for fluid flow. However, in the case where the fault is reactivated, the subsequent brittle deformation may in turn result in a porosity increase (Figures 3I,J; e.g., Grare et al., 2018).
Despite the dissolution/precipitation processes, which cause the most common problems within sandstones, another issue may be clogging of the wells or a reservoir rock by suspensions within the injected water (Figure 3E; e.g., Brehme et al., 2018). The source of these suspensions may be small mineral particles formed for instance by the oxidation of the steel pipelines, and/or precipitation of minerals caused due to lower pressure and temperature of the injected water compared with the groundwater (Su et al., 2018). Moreover, microbes, such as sulphate- and iron-reducing bacteria or saprophyte, may reproduce very quickly under suitable conditions, forming biofilms around the reinjection well and also cause clogging of the wells or a reservoir rock (Su et al., 2018). Fines migration, precipitation, biofilm, and corrosion lead to a skin effect around the wells and are the main causes for the exponential injectivity decline in sandstone geothermal systems (e.g., Brehme et al., 2018).
Carbonates
Deposition in varying environments and intricate diagenetic processes lead to the heterogeneity and complex microstructures of carbonate rocks, which often pose a significant problem when it comes to understanding and predicting their reservoir quality (e.g., Lønøy, 2006). At the same time, carbonate minerals are extremely reactive, thus they experience rapid rates of porosity reduction and c.50% of carbonate reservoirs have a porosity of <16% by the time they are buried to 750 m depth (Ehrenberg and Nadeau, 2005). Therefore, carbonates are likely to deform in a brittle-dilatant manner, forming faults and fractures acting as conduits to fluid flow (Kaminskaite, 2019). Hydraulic pathways in carbonates are therefore bound to fracture networks, faults and adjacent karstification and/or dolomitized zones (Figures 4E,F; e.g., Lopez et al., 2010; Niederau et al., 2015; Montanari et al., 2017; Wang Q. et al., 2019). The fault dip influences the circulation depth and, hence, the resulting water temperature, thus thermal springs often form along the faults (Li et al., 2007). Deep karstic aquifers containing hot water are the best targets for geothermal heat utilization in carbonates, UTES and CCS development due to their favourable characteristics, such as high single-well yield, low salinity, easy reinjection and fewer environmental impacts during utilization (Kong et al., 2014). Cavities are generally stable due to the favourable mechanical properties of carbonates thus the risk of collapse or subsidence is low (Goldscheider et al., 2010). The identification and location of hydraulically conductive zones is of special interest for geothermal reservoir prediction (Figure 4). However, identification of these high permeability zones in deeply buried carbonates is difficult, and despite the formation of brittle structures and karsts, reservoir permeability also depends on the hydrochemical conditions of the carbonate reservoir to maintain these open flow paths; this is a subject still poorly understood.
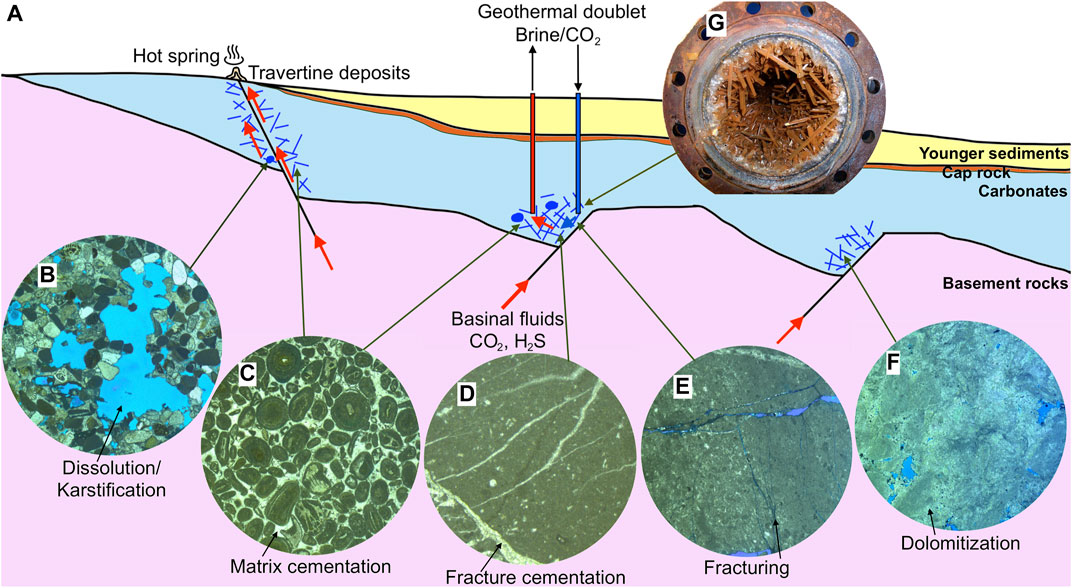
FIGURE 4. (A) Schematic illustration of a geothermal and/or CCS system in a carbonate reservoir, showing structural features and groundwater flow paths. Arrows indicate flow direction, and blue to red colours indicate cold and hot water, respectively. (B–F) Micrographs represent examples of products of the THMC processes that may occur within carbonates during the geothermal and/or CCS technology installation/operation (field of view: 10 mm): (B) Vugs (or karsts at a field scale) forming due to dissolution; (C,D) Cementation within the rock’s matrix (C) and fractures (D); (E) Open fractures; (F) Dolomitization that changes the rock’s structure (rhombic structure) and porosity (vuggy-intercrystal); relics of a carbonate limestone form dark fossil-shaped patches. (G) Photograph of a pipe cross-section (diameter: 219 mm), taken from a geothermal plant in Lithuania “Geoterma” showing gypsum precipitation (from Vaitiekūnas, 2012).
The most important diagenetic processes that may occur in geothermal reservoirs of carbonates are pressure solution, dissolution, dolomitization and cementation (Figures 4B–D). Dissolved CO2 reacts with calcite to form soluble and pH-neutral calcium and bicarbonate ions, thus dissolution processes in carbonates can form natural sinks for CO2 (e.g., Liu and Zhao, 2000). However, this process is generally considered minor as the highly reactive rock rapidly reaches chemical equilibrium unless flow rates are very high (e.g., Sanford and Konikow, 1989). In a closed system, calcite solubility is prograde up to 125°C and then becomes retrograde, while in an open system, calcite solubility is retrograde over this temperature range, meaning that calcite solubility increases with decreasing temperature (Wood, 1986). Therefore, more CO2 and carbonate dissolution will occur within the cold region that forms around the injection well, which will widen the fracture apertures and produce “inverted” karsts (Figures 4A,B; Andre and Rajaram, 2005). Dissolution by water or aggressive fluids may create extremely high-permeability layers (Schmoker and Halley, 1982; Brown, 1997). Moreover, the presence of acids may increase dissolution even more, for instance, oxidation of H2S creates sulphuric acid which boosts karstification (Palmer, 1991). However, in case of a decarbonisation technology installation dissolved calcite may migrate away from its source and precipitate as a cement in the adjacent rock (e.g., Garrison, 1981). Therefore, the amount of cement may vary both regionally and locally in response to flowing water through the sediments and the intricate dynamics of re-precipitation and/or dissolution/pressure solution at the local to regional scale.
Carbonate precipitation depends on several factors, such as temperature, rate of CO2 degassing and supply of Ca2+ and CO2−3 ions (Jones, 2017). In literature, different critical temperatures are suggested for calcite precipitation, varying from 40°C to 70°C (Kallesøe and Vangkilde-Pedersen, 2019). There are, however, cases where calcite dendrite crystals precipitated at temperatures >80°C (e.g., Jones et al., 2000), and Della Porta (2015) suggests that calcite may precipitate at temperatures <30°C. The lowest temperature for aragonite precipitation at hydrothermal vents is 40°C (Folk, 1993). In the Italian thermogene travertine deposition settings aragonite precipitates at vents where temperature is between 45 and 62°C despite the low Mg/Ca ratio (Della Porta, 2015). In distal areas of thermogene travertine systems, where water temperatures have cooled to <40°C, aragonite may precipitate when the Mg/Ca ratio in the residual fluid has increased due to progressive precipitation of low Mg calcite (Kele et al., 2008). Therefore, cold CO2/waste water injection into a reservoir may lead to undersaturation of calcite, inhibiting precipitation of carbonate minerals around the injection well (Figure 4G; Sigfússon and Uihlein, 2015). However, another factor controlling carbonate precipitation is the rate of CO2 degassing. Under the same temperature conditions, CO2 solubility decreases with pressure. Wood, (1986) suggests that temperatures <125°C and high CO2 pressures are most effective in mobilizing calcite. CO2 outgases away from the borehole resulting in decrease in CO2 pressure and hence reduced calcite solubility. That is the reason why calcite scale occurs near the flash point in the production wells where vapour is being released during flashing (Yanagisawa, 2015). Furthermore, as calcite solubility is lower at high temperature conditions, calcite precipitation tends to occur in mid-section or shallow areas of production wells where flashing occurs. Similarly, solubility of anhydrite is lower at higher temperature and tends to precipitate at deep points of production wells and at shallow high temperature points. Anhydrite scaling at high temperature zones in production wells is found in many geothermal fields, for instance, at Sumikawa geothermal field and Hijiori EGS test site (Kato et al., 2000; Yanagisawa, 2015).
Degassing of CO2-rich thermal waters causes precipitation of carbonate minerals such as the widespread travertine deposits in Pamukkale, Turkey (Brogi et al., 2014), and central Italy (Minissale, 2004) or the rich speleothems in the Buda Karst (Erőss et al., 2008) (Figure 4A). Mixing of reducing water from deep flow systems with oxygen-rich water from shallower flow systems may cause precipitation of iron and manganese oxides and hydroxides (Goldscheider et al., 2010). High concentrations of sulphate may also accumulate in the discharge zones of carbonate aquifers due to oxidation of sulphide minerals such as pyrite, or from the dissolution of gypsum and anhydrite, or due to deep fluids rich in hydrogen sulphide transforming into sulphuric acid when it comes in contact with oxygen-rich water (Goldscheider et al., 2010). The authors suggest a direct relationship between levels of sulphate and temperature, and an inverse relationship with discharge. Microbial mats are also associated with carbonate precipitation along hydrothermal springs (e.g., Casanova et al., 1999).
Upward migration and diffusion of hydrothermal fluids along faults in carbonates may also cause silicification, where calcite/aragonite/dolomite is replaced with opal/chalcedony/low- temperature quartz (Menezes et al., 2019). Hydrothermal silicification can greatly increase the porosity and permeability of carbonate geothermal reservoirs by forming mm-cm-scale vugs (Packard et al., 2001; Poros et al., 2017; You et al., 2018; Lima and De Ros, 2019; Menezes et al., 2019). Silicified zones in carbonate lithologies are typically thicker than in siliciclastic rocks, where unlike carbonates, silicification results in reduced porosity compared to the host rock (Menezes et al., 2019).
Granite
Granite is a hard, massive, coarse grained igneous crystalline rock with generally low permeability and isotopic physical parameters. However, in practice, it often contains a wide range of structures at depth, including faults, mineral filled fractures, and open or mineral-bridged fractures (e.g., Segall and Pollard, 1983). Granite is rich in elements with heat-producing radioactive isotopes (K, Th, U), and is thus commonly associated with temperature anomalies and elevated geothermal gradients within the crust, which makes it a suitable geothermal reservoir rock (e.g., Sliaupa et al., 2010; Shao et al., 2015). Granite consists of quartz, plagioclase and alkali feldspars, and smaller amount of biotite, muscovite and hornblende (Farndon, 2010). These minerals have different thermal expansion coefficients and thermo-elastic characteristics (Zhu et al., 2018), but are stable under dry conditions and temperatures of up to 300°C (Okamoto et al., 1991). The mineral composition of granite influences its strength significantly, because cracks propagate at the weakest planes within the rock (Shao et al., 2015). Depending on temperature, thermal cracking may occur either between adjacent crystalline grains in some of the weaker mineral constituents, such as feldspar and biotite grains (intergranular cracks) (Brace et al., 1972), or within grains (intra-granular cracks) (Glover et al., 1995).
Kumari et al. (2017) suggested that at temperatures <300°C, the effect of depth/confining pressure is much greater on the mechanical behaviour of granite than temperature. Takahashi and Hashida, (2004) performed experiments on granite and showed that the strength of the granite under air-dry conditions is temperature insensitive within the studied temperature range (up to 600°C) and nearly constant up to 350°C under water-saturated conditions. However, under temperatures above the critical point of water (>374°C and 22 MPa), the strength decreased rapidly with increasing temperature. That is due to supercritical water enhanced stress corrosion cracking, which may further enhance the reservoir permeability (Takahashi and Hashida, 2004). At temperatures >400°C, some minerals, such as illite and kaolin, may be decomposed and volatilized (Hu et al., 2018). Oxidation/decomposition reactions producing minerals such as ankerite, siderite, magnetite and pyrrhotite are evident in the temperature range of 400–600°C (Hu et al., 2018). However, this range of temperatures is currently not reached in the geothermal energy systems.
Lo Réet al. (2014) observed two common dissolution/precipitation reactions occurring in granites during hydrothermal experiments: 1) feldspars are the most reactive primary minerals, thus they are the first to dissolve or be altered (Figures 5C,D), and 2) regardless of temperature, common secondary mineral precipitation includes smectite, mixed-layer clays, illite, zeolite, and silica with fewer occurrences of kaolinite, anhydrite, calcite, chlorite, albite, and K-feldspar (Figures 5B,C,E–G).
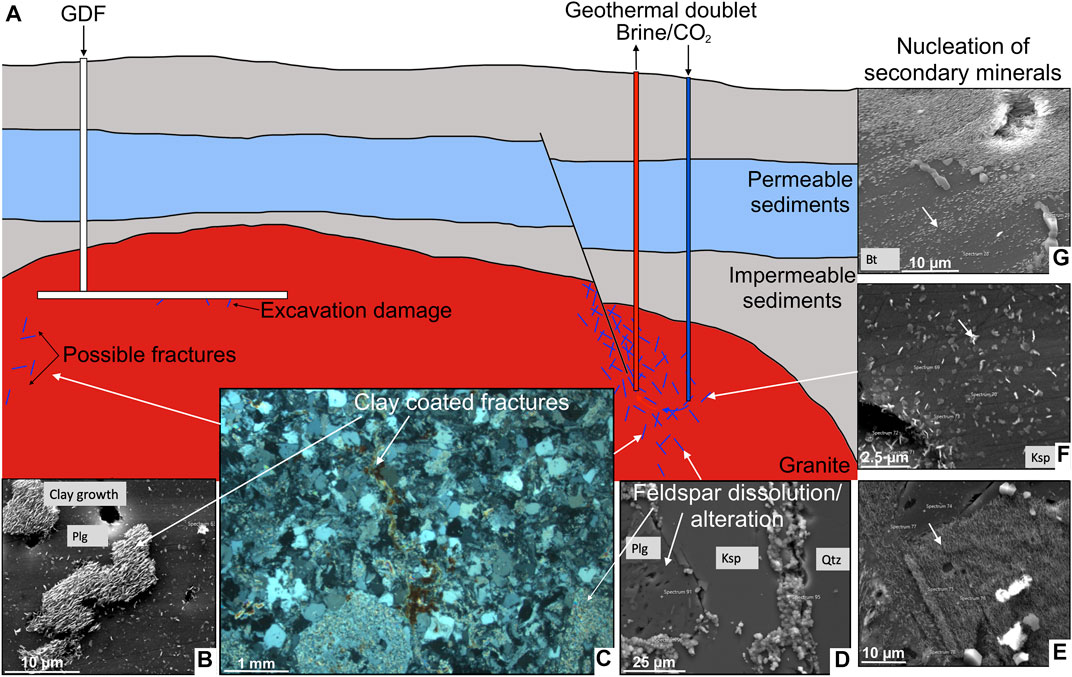
FIGURE 5. (A) Schematic illustration of a geothermal, CCS and nuclear waste disposal system in a granite reservoir, showing structural features and groundwater flow paths. Arrows indicate flow direction, and blue to red colours indicate cold and hot water, respectively. (B, D–G) SEM images and (C) a micrograph that represent examples of products of the THMC processes that may occur within granites during the installation/operation of shown technologies: (B,C) clay-coating on fracture walls; (C,D) feldspar dissolution/alteration; (E–G) nucleation of secondary minerals (biotite, different types of clays).
Fractures provide essential fluid pathways in granitic geothermal reservoirs due to their extremely low matrix permeability (Figure 5A). Natural fluid circulation within hydrothermally altered and fractured zones in granites shows strong hydrothermal vein alteration with clay mineral deposition (illite) and many secondary minerals (quartz, calcite, ankerite, dolomite, clays, pyrite, hematite, etc.) (Genter et al., 2000; Genter et al., 2016). Fresh meteoric water was circulated through a jointed granite reservoir in the Rosemanowes test site in the UK at a depth of 1.6–2.6 km at initial rock temperatures of 58–100°C (Richards et al., 1992). The authors presented geochemical data from selected circulation experiments over a period of 8 years. Early tests showed waters depleted in K, Ca and Mg, and enriched in Na, SiO2, CI and alkalinity. Later tests showed depletion in Mg, enrichment in Na, Ca, SiO2, CI and alkalinity. Various processes that might have given rise to these changes were considered, including diffusion from saline pore fluid, cation exchange, mineral dissolution and precipitation, as well as bacterially-mediated reactions. The major salinity-generating processes in the reservoir were inferred to have been the diffusion of Cl salts from saline pore fluids into the injection water and the generation of HCO3 by bacterial oxidation of dissolved and particulate organic matter. The cation exchange sites were inferred to be on natural clay minerals coating the joint surfaces, whereas additional clay minerals may have come from plagioclase dissolution during water circulation (Figures 5B,C; e.g., Miller et al., 2000). The principal evidence of dissolution reactions in the early tests was the release of SiO2. Other solutes were inferred to have been largely controlled by cation exchange.
The extremely low permeability and high strength of massive granite make it a good potential storage site for nuclear waste (Yoshida et al., 2000) (Figure 5A). However, possible fractures and oxidizing groundwater moving through them represent a potential hazard for dispersion of radionuclides (Dideriksen et al., 2010). Radionuclides may become physically trapped in fractures due to the existence of pore spaces between the surface of a fracture filling and the host rock matrix (Yoshida et al., 2000). Clay in fractures may act as retention sites for radionuclides by sorption (Missana et al., 2006). Trapped radioelements may be released when renewed circulations of hydrothermal solutions initiate the dissolution of the secondary mineral species responsible for the trapping (Ménager et al., 1992).
Shales/Mudstones/Claystones
Shales/mudstones/claystones (later in the text referred to as shales) exhibit a wide range in rheology depending on a range of factors including porosity, mineral composition, organic matter content and stress history (Okamoto et al., 1991). High porosity, clay-rich, normally consolidated shales often deform in a ductile manner whereas lower porosity shales containing high volumes of quartz and/or carbonate deform in a more brittle manner (Figure 6A). This deformational behaviour is critical to understand because it controls not only whether faulting increases or decreases permeability but also how faults and fractures reseal. Typically, shales contain substantial amounts of clay minerals, quartz, carbonates, and smaller quantities of feldspars, iron oxides and organic matter (Shaw and Weaver, 1965). Clay composition is also very important, as, for instance, illite clays are non-expanding clays because the K, Ca, or Mg interlayer cations prevent the entrance of H2O into the structure, whereas weak linkage by cations (e.g., Na+, Ca2+) in smectite clays results in high swelling/shrinking potential (Lagaly, 2006). The clay minerals present in shales are largely kaolinite, smectite/montmorillonite, chlorite and illite (Shaw and Weaver, 1965). Minerals and pore space in shales have a strong preferred orientation within the bedding plane due to the sheet-like structure of the clay minerals (Figures 6D,I; Ougier-Simonin et al., 2016). The most important THMC processes that are likely to occur in shales during decarbonisation technology applications are:
(1) Clay hydration/swelling. Clay hydration is a physical process that takes effect immediately in contact with aqueous solutions resulting in clay swelling (Figure 6F; e.g., Anderson et al., 2010). Swelling effect of clays may cause a huge reduction in pore space, which not only reduces matrix permeability, but the swelling of clay contents along the fracture surfaces may also result in fracture closure (Zhang et al., 2017). However, the increased volume of plastic clay material separates the contacts between the stronger quartz particles, weakening the shale formation and enhancing its ductile properties (e.g., Pineda et al., 2014; Ougier-Simonin et al., 2016). Because of the clay minerals affinity to absorb water and to swell, shales are more prone to water weakening than other rock types (Chen X. et al., 2019).
(2) Dehydration and thermal shrinkage. Dehydration of swelling clay minerals occurs when shales are subjected to shrinkage and volume reduction, during which clay will release water (Guo et al., 2014). In the immediate vicinity of the heat sources, as in the case of nuclear waste repository, drying out of the clay may occur if water was able to migrate and/or to vaporize. This drying-out would induce shrinkage of the clay, resulting in some degree of fracturing (Okamoto et al., 1991). In laboratory experiments, as more and more hydration/dehydration cycles are performed, the thermo-chemically induced microfractures in illite open wider, while in smectite the microfractures heal during hydration, except when they interact with a hard mineral (Montes-Hernandez et al., 2004).
(3) Decomposition of organic matter. During shale formation, fluids may be generated as the organic matter matures, causing local volume increases with resultant anomalously high pressure. These overpressures locally lower the effective overburden stress, causing microfracture development (e.g., Padin et al., 2014). However, most organic-rich shales are oil-wet, which means that hydrocarbons can escape quite rapidly without having to overcome a capillary pressure (Brown, 2000). The overpressure that is developed tends to be controlled by the half bed thickness, the permeability and more importantly the capillary entry pressure of the units lying above the oil-wet source rock. Where expulsion of petroleum is not retarded by low rock permeability, high pore pressure does not develop, organic porosity compacts and kerogen shrinks, and hence the source rock thins, causing fracturing (Brown, 2000). Maturation of kerogen and decarboxylation of organic matter may release CO2 (Chen B. et al., 2019).
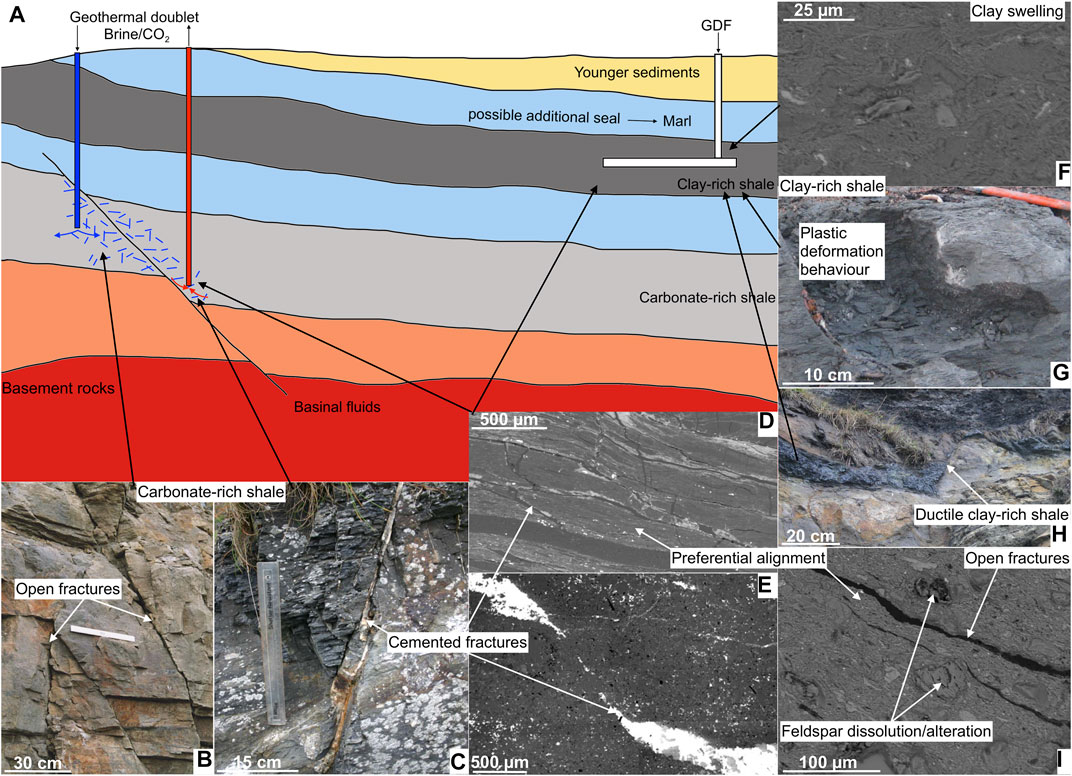
FIGURE 6. (A) Schematic illustration of a geothermal, CCS and nuclear waste disposal system in shale-mudstone reservoirs, showing structural features and groundwater flow paths. Arrows indicate flow direction, and blue to red colours indicate cold and hot water, respectively. Note, that nuclear waste disposal is illustrated within the clay-rich shale, whereas geothermal doublet and CO2 injection wells are shown to use the carbonate-rich shale. (B,C,H,G) are outcrop images and (D–F,I) are SEM images that represent examples of products of the THMC processes that may occur within shales/mudstones during the installation/operation of shown technologies: (B–E,I) brittle deformation forming open fractures (B,I) and cemented fractures (C–E); (F) clay swelling; (G,H) ductile deformation; and i) feldspar dissolution/alteration.
Fractures in shales may also form due to differential compaction, local and regional stress changes, strain accommodation around large structures and uplift (Gale et al., 2014, and references therein). Fractures formed by any of the above processes may close due to several reasons, including a local change in stress state, inelastic deformation of the matrix, and precipitation of minerals (Ougier-Simonin et al., 2016). Groundwater or hydrothermal fluid flow present in the fractures may precipitate minerals such as calcite, quartz and pyrite (Figures 6C–E; e.g., Zeng et al., 2013). Hydrocarbons, including viscous bitumen, may also fill these fractures (Lash and Engelder, 2005). Subsurface fractures found in core are most commonly sealed, but barren fractures are also present in some cores, even though their origin is often uncertain (Gale et al., 2014, and references therein). The tensile strength of the contact between the sealing mineral and the shale wall rock is often low, thus the fracture-host boundary is weak and new fractures may propagate preferentially at their interface (e.g., Zeng et al., 2013; Lee et al., 2015). Precipitates may also act as proppants between fracture walls or by generating sufficient crystallization stress to induce tensile fracturing in the surrounding rock (e.g., Hilgers and Urai, 2005; Menefee et al., 2020).
A rise in temperature generally reduces the swelling capacity of clay minerals (Villar and Lloret, 2004; Chen et al., 2020). At elevated temperatures, subcritical fracture growth can be significantly enhanced in all types of shales, indicating an increase in fluid diffusion rates from the fracture into the matrix and thus enhanced chemical weakening in the fracture process zone (Chen et al., 2020).
Injection of dry supercritical CO2 into subsurface reservoirs would result in a local increase in water salinity which would in turn inhibit clay minerals from hydrating and hence reduce the risk of subcritical fracture growth in clay-rich shales and likely enhance their sealing performance (Chen X. et al., 2019). Consequently, injection of water that is less saline than the resident brine may facilitate fracture growth across clay-rich shales, whereas injection of more saline brine would increase strength. Injecting low-salinity brine dilutes the electric double layers (EDLs) between the clay particles, raises the negative charges of clay surfaces, and consequently strengthens the repulsive forces in-between (Khishvand et al., 2019). This process may expand the EDLs, change the established equilibrium, and ultimately detach some of the mixed-wet clay particles from the solid surface, enhancing permeability of the shale reservoirs.
In carbonate-rich shales, acidification of water through the dissolution of CO2 increases the propensity for subcritical failure (Chen X. et al., 2019). This agrees with the observation that moderate amounts of calcite dissolution aids fracture growth in carbonate rocks (Atkinson, 1984), while excessive dissolution may lead to fracture tip blunting and suppress fracture propagation (Rostom et al., 2013). However, carbonate minerals have fast reaction dissolution and precipitation kinetics thus reactive fluids can promote near-immediate and extensive precipitation (e.g., Menefee et al., 2020), which buffers solutions keeping the pH fairly constant. Major et al. (2018) showed that shale in the damage zone affected by hydrothermal springs has higher fracture toughness, and hence, strength, compared to unaltered shale, which the authors attributed to calcite cementation.
Clay-rich shales are good potential sites for nuclear waste repositories, because of their very low hydraulic conductivity and potential for self-sealing (Tsang et al., 2012). Moreover, as mentioned in Granite section, clay minerals also provide good sorption capacity for the retardation of radionuclide transport. Therefore, ductile clay-rich shales are more preferred sites for nuclear waste storage, whereas brittle carbonate-rich shales are more suitable for geothermal systems (Figures 6A,B).
Evaporites
Evaporite deposits are of sedimentary origin and form by precipitation of various salts from evaporating water, such as seawater. The main evaporite rocks are gypsum, anhydrite and halite, however potash and other rarer salts are also locally important (Martinez et al., 1998). Evaporites may form hundreds of meters thick layers and are often interbedded with other country rocks, such as limestone, dolomite and shale (Figure 7A). Evaporites are the most soluble rocks and their dissolution often forms the same types of karst features found in carbonates. The only difference is that karsts in evaporites form rapidly, within days, weeks or years, whereas karsts in carbonates typically form in years, decades or centuries (e.g., Johnson, 2007; Zidane et al., 2014). Salt caverns are perfect short-term sites for CO2, hydrogen and compressed air energy storage because they can provide large volumes of space (Figure 7A; e.g., Shi and Durucan, 2005; Lankof et al., 2016).
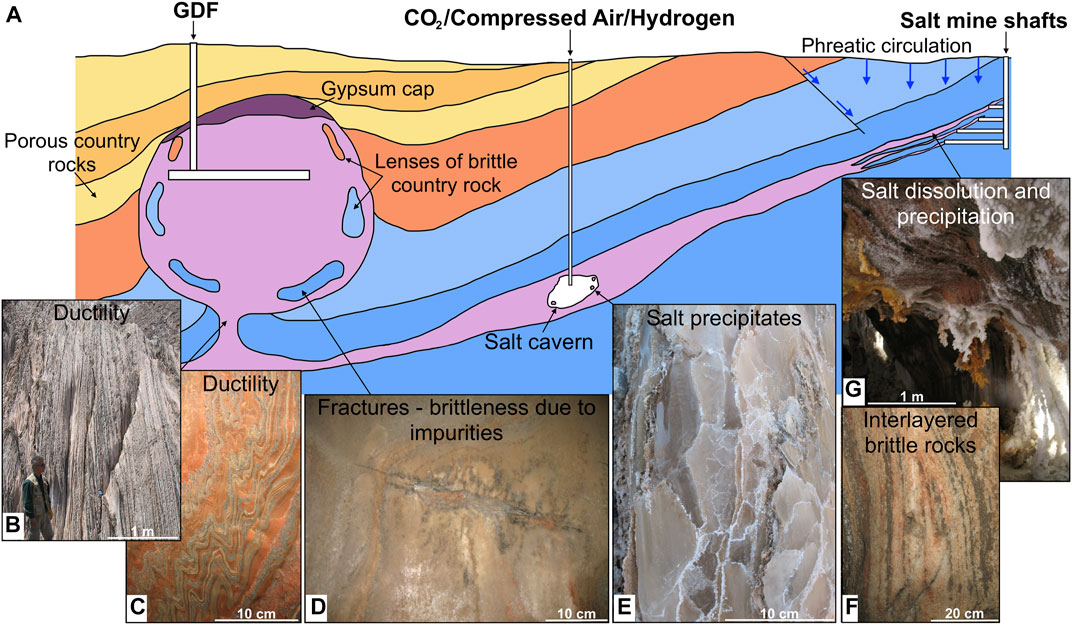
FIGURE 7. (A) Schematic illustration of the CCS, hydrogen storage, CAESC and nuclear waste disposal systems in evaporite reservoirs, showing structural features and groundwater flow paths. Blue arrows indicate groundwater flow direction. (B–G) are outcrop images (Cardona salt diaper, Spain) that represent examples of products of the THMC processes that may occur within evaporites during the installation/operation of shown technologies: (B,C) ductile deformation; (D,F) brittle deformation due to salt impurities; (E,G) salt precipitation; and (G) salt dissolution.
Salt can act as an excellent seal, as evidenced by its ability to hold back significant columns of highly overpressured fluids (Warren, 2017). Evaporite seals, with their high entry pressure, superior ductility, very low permeability and large lateral extent, tend to maintain excellent seal integrity over vast areas, even when tectonised and exposed to a wide range of subsurface temperature and pressure conditions (e.g., Macgregor, 1996). Salt beds tend to leak when thinned, dissolved, drilled and contain higher levels of non-salt impurities (Warren, 2017).
Salt may flow over geological time resulting in diapiric structures known as salt domes (Heroy, 1957). Salt domes/diapirs often contain one to 10 m thick lenses of other country rocks embedded during salt flow (Figure 7A). These lenses are brittle, representing the main problem during salt mining and waste disposal as they may acquire open fractures forming pathways for fluid flow (Figures 7D,F; e.g., Warren, 2006). However, these fractures only become important if they connect to the surrounding rock outside the salt.
Gypsum is the most widespread evaporite mineral to form in near surface environments. Gypsum converts to bassanite, which is metastable and decomposes to anhydrite when buried to temperatures greater than 64–85°C (Murray, 1964; Yamamoto and Kennedy, 1969). The conversion of gypsum to anhydrite reduces the volume by 40% and releases much water, which results in rheological weakening and mechanical destabilization of evaporite bodies (Urai et al., 1986). It also causes formation of chemically aggressive brines, which makes gypsum inappropriate for any kind of radioactive waste storage (Borojević Šostarić and Neubauer, 2012).
Anhydrite remains metastable in either under- or supersaturated solution due to the very slow dissolution and growth kinetics of anhydrite at temperatures lower than 80°C (Van Driessche et al., 2017). However, anhydrite is brittle and may contain open fractures. Anhydrite is potentially good storage stratum for low-/intermediate level radioactive waste but only when fulfils the following requirements: 1) located above groundwater level, and 2) a seal is present both at the top and base to protect the anhydrite layer from water inflow (Borojević Šostarić and Neubauer, 2012).
Halite is thermally stable over the range of temperatures expected in radioactive waste repositories (Borojević Šostarić and Neubauer, 2012). Halite has a very high ductility and ability to stream, reanneal and re-establish widespread lattice bonding via pressure-solution creep and dislocation creep, which give it a low susceptibility to fracturing (Figures 7B,C; e.g., Warren, 2017). Disadvantages of halite are the high heat conductivity and high solubility in water. Locations in arid climates are thus suggested by Borojević Šostarić and Neubauer, (2012).
Solubility of halite increases with temperature (e.g., Blanco-Martín et al., 2018). Dihedral angle of halite is also a thermodynamic property that changes with pressure and temperature (Warren, 2017). The solid-solid-liquid interfaces of polyhedral halite remain sealed when the dihedral angle is >60°, which is typically a case at mesogenetic temperatures (e.g., Lewis and Holness, 1996). At burial temperatures of >100°–150°C and pressures of >70 MPa, polyhedral grain boundaries may attain dihedral angles of <60°. Fluid inclusion filled intercrystal cavities may then link up, and the salt mass can become permeable (Warren, 2017), losing its ability to act as a seal (Lewis and Holness, 1996).
The ability of salt to flow also increases with increasing temperature (Okamoto et al., 1991). Therefore, in the vicinity of the heat sources, the creep of salt will result in rapid closure of the disposal holes and thus a restoration of the confinement pressure on the waste packages. Crushed salt may thus be used as a backfill material in the GDF. With time, it will recrystallize creating mechanical and flow properties that will evolve toward the characteristic values of the natural salt host rock, providing seal properties (Martin et al., 2015).
Salt generally contains very little water, <0.3% in volume in diapiric salt and slightly higher in bedded salt (Okamoto et al., 1991). Inclusions of brine in rock salt tend to migrate towards the heat sources if the thermal gradient is sufficiently high. Evaporation of brine near the heat source triggers precipitation of salt and hence the reduction in permeability (Figure 7E), whereas condensation of moisture in cooler areas leads to the dissolution of salt and increases in permeability (Olivella et al., 2011; Blanco-Martín et al., 2018). Dissolution/precipitation reactions resulting from evaporation and condensation of brine may strongly affect fluid flow to and around the nuclear waste canister (Olivella et al., 2011; Bourret et al., 2017).
Warren (2016) gives several reasons why the existing salt mines are not the most suitable places for a low to medium level nuclear waste storage. Firstly, he argues that all salt mines are shallow (<1.1 km), thus circulation of subsurface/phreatic waters are likely (Figure 7A). Secondly, mining operations often continue in a particular direction along an ore seam until the edge of the salt is intersected with the high fluid transmission zone, thus they have a history of flooding. Thirdly, bedded halite beds are thin (>10–50 m) and typically interlayered with laterally extensive brittle carbonate, anhydrite or shale beds. Therefore, Warren (2016) suggests that the depth range of 1–2 km is the most ideal for storage in salt cavities because: 1) cavities located deeper than 2 km are subjected to compressional closure or salt creep; 2) cavities shallower than 1 km are subjected to the effects of deep phreatic circulation which would lead to large-scale dissolution (Figure 7G).
Crustal regions above salt formations might be suitable geothermal reservoirs due to the high thermal conductivity of a salt rock, that causes local positive thermal anomalies in the overburden of salt accumulations (Norden and For̈ster, 2006; Moeck, 2014).
Coal
Coal is a combustible sedimentary rock, formed as a rock strata called coal seams (Figure 8A). Coal is largely made up of carbon but it also contains small quantities of the non-organic compounds like quartz, clays, pyrite, carbonate and other elements, such as sulphur, hydrogen, oxygen and nitrogen. For 20 years, CO2 has been injected into coal seams to enhance the recovery of methane (e.g., Ranathunga et al., 2017). The displaced methane is produced through an in-situ desorption process, whereas the adsorbed CO2 becomes permanently stored within the coal: CO2/CH4 sorption ratio varies from 1.1 to 9.1 (Busch and Gensterblum, 2011, and references therein). Therefore, the enhanced coalbed methane (ECBM) recovery technique is considered as a potential approach to CCS in deep coal seams (Figure 8; e.g., White et al., 2005; Shi and Durucan, 2005). One of the main problems associated with developing ECBM is the low permeability of most coals (Lokhorst and Wildenborg, 2005). Open cleats in coal can provide the required pore space. They usually occur in two sets that are mutually perpendicular and also perpendicular to bedding mostly due to compaction, desiccation of peat (coalification), tectonic events, unloading effects and progressive devolatilization reactions (Figure 8E; Laubach et al., 1998). CO2 injection into the coal mass causes it to swell, leading to significant alterations in its internal rock mass structure and thus major modifications in its strength properties and reductions in permeability and hence injectivity (Ranathunga et al., 2017). Therefore, CO2 stream needs to be mixed with other gases, such as nitrogen, to supress swelling (Grattoni et al., 2016). Adsorption of CO2 is stress dependent (e.g., Gensterblum et al., 2014) and very little work has been done to assess whether cycling of injection of different gases could increase methane desorption and increase CO2 adsorption.
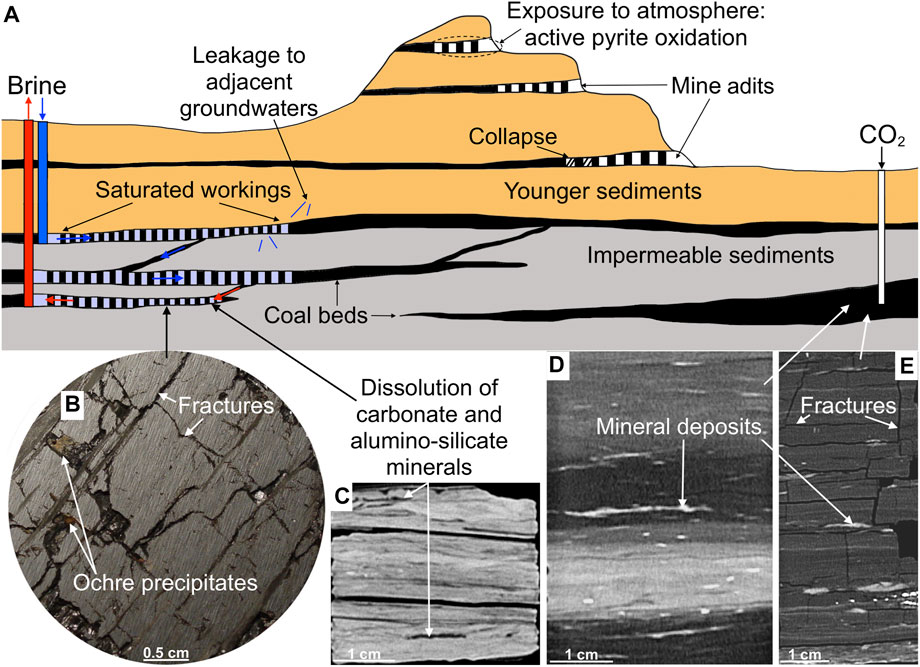
FIGURE 8. (A) Schematic illustration of the geothermal heat extraction, heat storage and CCS systems in coal mines and deeper coal seams, showing structural features and groundwater flow paths. Arrows indicate flow direction, and blue to red colours indicate cold and hot water, respectively. (B) Photo of a core plug surface and (C–E) CT-scan transects across core plugs that represent examples of products of the THMC processes that may occur within coal during the installation/operation of shown technologies: (B) precipitation of ochre; (C,D) mineral precipitation/dissolution; and (B,E) open fractures.
Mine water in abandoned and operating coal mines is also recognized as a potential source of geothermal heat and energy and/or a place for heat storage (Figure 8A; e.g., Hall et al., 2011; Loredo et al., 2017; Banks et al., 2019). There are several examples in the world, which currently utilize geothermal coal mine water. For instance, mine water heat recovery schemes are implemented and used for space heating at the National Coal Mining Museum in Wakefield, UK, with a temperature of 14.5°C (Athresh et al., 2016), Markham Colliery in Derbyshire, UK, at 15.4°C (Athresh et al., 2015), Park Hills, Missouri, at 14°C, and Shettleston, Scotland, at 12°C (Hall et al., 2011).
Each coal mine groundwater system is unique and consists of a number of aquifers with varied geochemical characteristics. Mine waters from apparently similar mine types can be highly acidic or alkaline, depending on the complex interplay of hydraulic, chemical and biological processes. Mine waters from greater depth tend to have higher conductivities due to longer rock-water interaction, greater potential influence of saline waters, and inflows of strata water with higher conductivity (Bailey et al., 2016). Therefore, the level of mineralization increases in groundwater with increasing depth of burial (Qian et al., 2016). Higher salinity and iron concentrations of deeper waters may pose a risk of operational problems with heat pumps, such as corrosion, encrustation or blocking of heat exchangers (Preene and Younger, 2014). Encounter with bodies of stagnant deep groundwater or dewatering of coastal mines and intrusion of modern seawater may cause leakage into coal mines causing critical safety issues (Qian et al., 2016), and potentially resulting in contamination of fresh surface water or groundwater with chloride (e.g., Headworth et al., 1980). Hydrochemical parameters can provide information about recharge and discharge sources of aquifers, and hence allow evaluation of aquifer connectivity and the sources of groundwater flow.
Mining allows the introduction of oxygen to the deep geological environment and thus the oxidation of minerals which are in a reduced state (Banks et al., 1997). The biggest problem related to oxidation is posed by ferrous iron present in mine water solution, which oxidises and forms ochre (Figure 8B), causing clogging of pipelines (Banks et al., 2019). Ochre precipitation may be avoided by keeping systems under positive pressure, limiting dissolution of oxygen. Oxidation of pyrite increases acidity and may dissolve minerals, such as marine apatite, containing radioactive material and heavy metals (Banks et al., 1997). However, in addition to posing an environmental threat through water and ground contamination, ochres can also act as a remediation material, trapping elements such as selenium and providing a unique source for its use as a commodity (Bullock et al., 2019).
While oxidation of pyrite within coal strata generates acidity, dissolution of carbonate minerals, such as calcite, dolomite, ankerite and siderite, usually in strata overlying the coal mine workings, serves to buffer acidity (Figures 8C,D; e.g., Younger, 1995). Dissolution of alumino-silicate minerals, such as olivine, pyroxene and anorthitic plagioclase, or feldspars and clays, even though only rarely found near coal, could also make contribution to neutralisation of pH (Banks et al., 1997). Therefore, the most net acidic waters tend to be derived from unsaturated workings with free access to oxygen, whereas more net alkaline waters are derived from more saturated or overflowing workings (Banks et al., 1997).
The oxidation of pyrite in mine drainage waters may also be catalysed by the action of acidophilic sulphide-oxidising bacteria, which thrives at a pH range of 1.5–3 (Banks et al., 1997). The authors note that Thiobacillus ferrooxidans, a chemoautotroph, derives energy for its metabolic processes from the oxidation of reduced sulphur and iron compounds and utilises CO2 as a carbon source. By catalysing the oxidation of ferrous sulphide to ferric sulphate, this bacterium greatly promotes the oxidation, hydrolysis and ochre formation (e.g., Hedin et al., 1994).
Pumping from flooded mine workings for geothermal purposes will potentially change the water pressures, and direction and velocity of water flow within the workings (Preene and Younger, 2014). This could lead to scour, instability or even collapse of existing underground mine workings. If boreholes are drilled into workings for the purposes of water extraction/injection this can also have a destabilising effect. Stability of mineshafts for minewater heat recovery depend on both water level and temperature fluctuations (Ng et al., 2019). Another potential issue in geothermal heat extraction from coal mines is thermal breakthrough of cool, reinjected water into the abstraction shaft, thereby producing cool water instead of the desired warm water. To avoid thermal breakthrough there should not be a direct connection between the injection and extraction boreholes (Banks et al., 2019).
Lithologies and Decarbonising Technologies: Discussion of Challenges and Opportunities
General Changes to the Physical State of the Subsurface Associated With Decarbonising Technology Application
It is evident that the most common result of THMC processes occurring within the subsurface caused by decarbonisation technology is fracturing changing the material properties significantly. The actual processes leading to fracturing differ in each scenario and may be the direct effect of the technology application, or occur due to the temperature change caused by it. It may also be created during excavation associated with technology deployment, forming damage zones around the wells and GDF excavation tunnels (e.g., Tsang et al., 2005, 2012). Fracturing may pose a significant risk, such as leakage of CO2 to the surface in CCS applications (e.g., Vilarrasa and Rutqvist, 2017), or radionuclide release to groundwater in the nuclear waste disposal systems (e.g., Benbow et al., 2014). However, fracturing may also be welcomed or triggered on purpose, like in the geothermal and CCS reservoirs, where enlarged network of fractures leads to an increase in hydraulic efficiency and storage volume of the reservoirs (e.g., Sigfússon and Uihlein, 2015).
Other common physiochemical changes are caused by precipitation-dissolution reactions, leading to corrosion and scaling of pipelines (Figure 4G) and other components of the decarbonising technology, cementation within the reservoir (Figures 4C,D) and hence reduction of the reservoir quality, as well as subsurface collapse in case of dissolution of large volumes of reservoir rock (Figure 4B). Precipitation-dissolution reactions depend on the pH change of the host rock and the formation water, temperature changes and/or oxygen entrapment into the system (e.g., Wood, 1986; Banks et al., 1997; Su et al., 2018). Each technology has a different effect on the solution pH, whereas oxygen may be introduced into any of the systems (Figure 9).
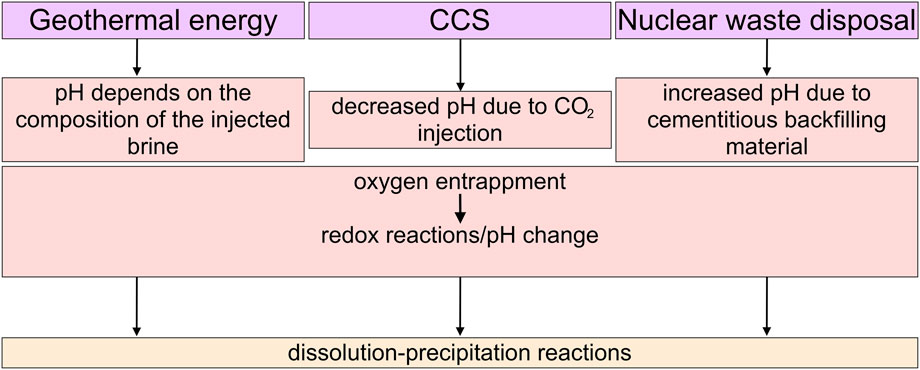
FIGURE 9. Schematic illustrating the effect of each decarbonising technology application on the solution pH of the host rock and the formation water, that may lead to various dissolution-precipitation reactions and with that the physiochemical state of the reservoir.
Reservoir Characterization for Decarbonisation Technology Evaluation: Importance of Considering the Effect of Physiochemical Processes and Their Link to Particular Reservoir Lithologies
In Lithology-Specific Processes Associated With Decarbonisation Technology Installations section, THMC processes are listed that are likely to occur in a specific lithology during decarbonisation technology application. However, rock type classification does not always have exact guidelines, thus the same rock often may be classified as several different rock types depending on the interpreter. For instance, tight sandstone with high clay content may be classified both as a sandstone and a shale. Moreover, problems associated with shales, such as clay swelling (e.g., Anderson et al., 2010) or mobility of clays due to electrostatic forces (e.g., Wilson et al., 2014), are also likely to occur in sandstones. Rocks with a high carbonate content (e.g., carbonate-rich shales, calcite-cemented sandstones) may have similar problems to those occurring in carbonate rocks, such as carbonate mineral dissolution and precipitation (e.g., Liu and Zhao, 2000). Therefore, mineralogy is one of the most important properties to assess before the reservoir evaluation, so that the other parameters and likely THMC processes could be predicted/simulated more accurately (Table 2). Thickness of beds, heterogeneity, fault and fracture network and geometry, confining stress and pore pressure are important reservoir properties for most of the lithologies when evaluating their hydraulic behaviour. Characterisation of the formation water properties, such as salinity, geochemistry, temperature and solution pH, is also crucial in determining the potential chemical reactions within the decarbonisation systems. However, such properties and parameters may have a strong effect on a reservoir performance in one lithology but show lower extent of impact in the other. Therefore, key reservoir characteristics and properties that are important in considering during initial feasibility screening and for the 3D geological and THMC simulation modelling for different types of lithologies are summarized in Table 2.
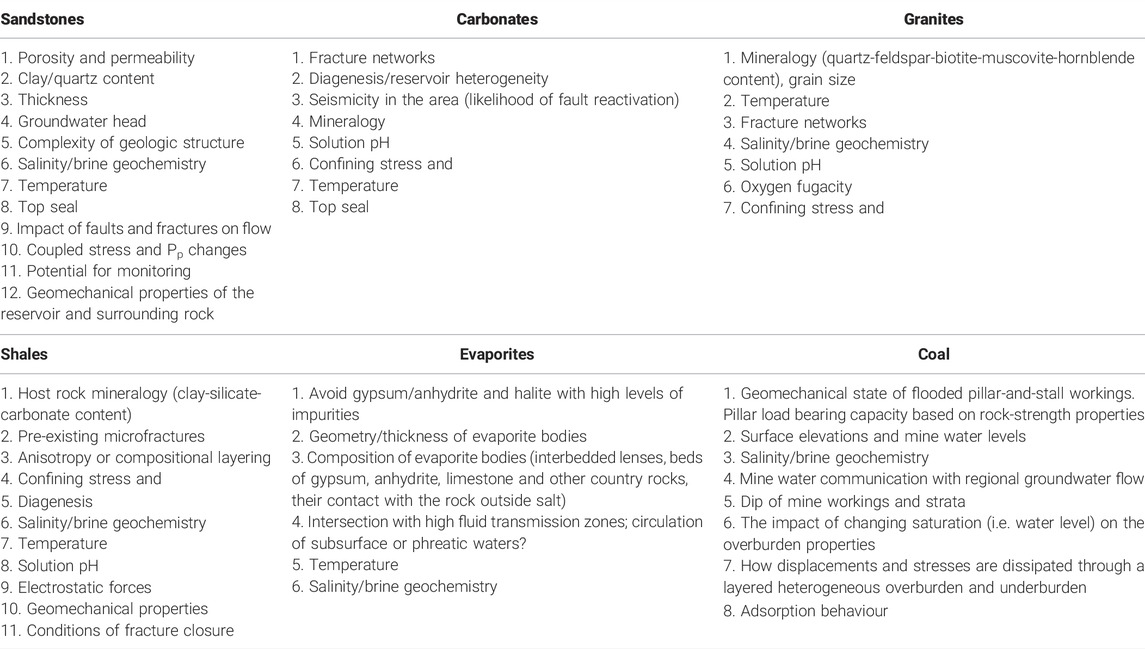
TABLE 2. Key parameters/properties which have the strongest effect on specific rock type reservoir performance and should be considered during reservoir rock evaluation for decarbonising technology installation.
Suitability of Different Lithologies for Particular Decarbonising Technologies
Geothermal Heat and Energy Extraction and Heat Storage
Table 3 qualitatively compares all key lithologies used for geothermal heat and energy extraction and heat storage in terms of their suitability for a particular decarbonizing technology. It is evident that only sandstones may have high enough matrix permeability to make them a viable hydrothermal reservoir rock for these technologies (Figure 3). Other lithologies have to be fractured, either naturally or artificially, to make these reservoirs productive (Figures 4–6). However, high mechanical strength of carbonates, granites and carbonate-rich shales make them deform in a brittle manner. Therefore, fault zones in these lithologies may be suitable sites for geothermal heat utilization, because it is likely they would be forming conduits to fluid flow. Unsuccessful Offenbach GT1 and Bellheim GT1 wells drilled within the Muschelkalk and Buntsandstein formations in the Upper Rhine Graben in Germany showed that in the Upper Rhine Graben only fault zones yield sufficient permeabilities for economic success and target definition should be based on 3D seismic surveys to map and evaluate fault zones (Reinecker et al., 2019). Therefore, finding a reservoir with sufficient matrix permeability may be difficult, especially taking into account that some mineral precipitation and scaling is going to reduce this permeability even further (e.g., Brehme et al., 2017; Brehme et al., 2018; Brehme et al., 2019).
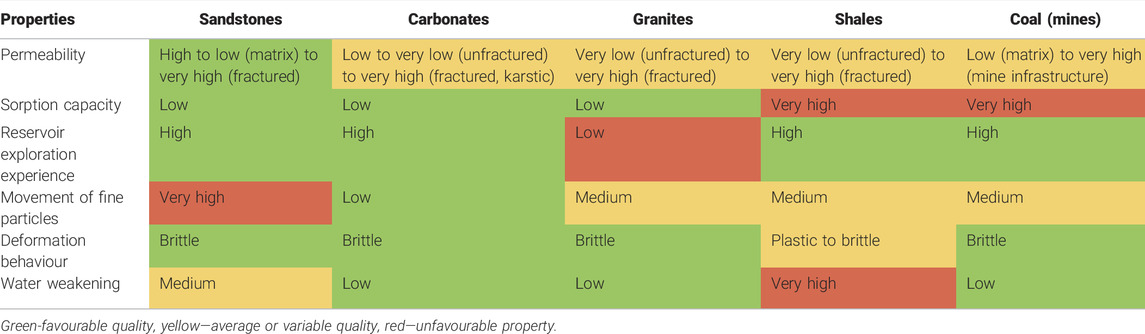
TABLE 3. Qualitative comparison of geologic media as a reservoir for geothermal heat and energy extraction.
Chemical reactivity of carbonates may increase their permeability by dissolution and dolomitisation reactions (e.g., Zhu et al., 2010; Biehl et al., 2016; Jiu et al., 2020). Dissolution often forms high vuggy porosity (Figure 4) or widespread karsts making carbonates one of the most attractive lithologies for subsurface utilization. Coal mine infrastructure is already available in many places around the world, providing very high permeabilities to make more sustainable use of low-enthalpy resources (Figure 8). Swelling may greatly reduce permeability in shales and coal, making the undamaged clay-rich and coal formations suitable rocks for sealing, but not great geothermal reservoirs. Mineral precipitation within the reservoir formation and scaling of wells is likely in any lithology (Figure 4G), especially the ones rich in chemically reactive minerals, such as calcite. The experiments done by Cui et al. (2017) on typical sandstone reservoir samples at temperatures >100°C showed that dissolution of ankerite and clay minerals can increase the concentration of Ca2+, Mg2+ and Fe2+ that can lead to the precipitation of silicate in the presence of CO2. For carbonate rocks, the increase of Ca2+ and Mg2+ caused by dissolution of dolomite can result in the precipitation of calcite and secondary ankerite. Simulations of geochemical reactions in CO2-water-rock systems indicate that for the sandstone reservoir, the reduction of the porosity caused by mineral precipitation has a negative effect on heat mining rate, while for the carbonate reservoir, the dissolution of dolomite and clay minerals can overshadow the precipitation effect of calcite and silicate minerals and increase the heat mining rate (Cui et al., 2017).
Clogging of the formation and pipelines due to suspension is most likely in sandstones due to the presence of fine particles, and it is least common in carbonates because grains in carbonates are well cemented and clay often makes a small proportion of the rock content (Table 3).
Working with geothermal reservoirs is very similar to working with hydrocarbons. Many techniques and needs are the same, only that hot water is the carrier of energy instead of hydrocarbons. Therefore, reservoir exploration and development experience gained in the oil and gas industry is applicable to geothermal heat and energy utilization, and skillsets should be transferred and not lost during the energy transition. Sandstone, carbonate and shale hydrocarbon reservoirs have been widely exploited. Records of some coal mine layouts may be available, and mine water treatment schemes are often applied after abandonment, making coal mines particularly easy to utilize (e.g., Athresh et al., 2015; Athresh et al., 2016). Granites are least explored in this respect. However, they are the only lithology considered here rich in elements with heat-producing radioactive isotopes, with granites often associated to anomalous geothermal gradients (e.g., Sliaupa et al., 2010; Shao et al., 2015). With each lithology having its advantages and disadvantages, it is important to assess the available geology in the area and the site-specific properties to determine which one is the most suitable for a given technology (Table 3).
Carbon Capture and Storage
Reservoirs for CCS have to fulfil similar criteria as those for geothermal energy: they have to have high porosity to be able to inject large amounts of CO2, and must be bound by impermeable rock units to prevent leakage (Table 4). However, CO2 has to be securely trapped, while geothermal water is extracted for usage. Moreover, CO2 flow is driven by buoyancy whereas water flows along a potentiometric surface. The fact that CO2 is a gas is also a big issue because it will expand to fill the volume available—water has low compressibility, thus it will not move unless there is a hydraulic head. Even though presence of a seal is crucial in both applications, long-term trapping mechanisms become extremely important in the CCS systems. Therefore, high sorption capacity is an advantageous rock property in this case, which is good in clay-rich rocks and coal. Even though evaporites show poor capacities for both sorption and mineral trapping, they are practically impermeable and form large cavities underground, that are stable in case of Earth movement or artificial damage due to its visco-plastic behaviour (Figure 7; e.g., Macgregor, 1996). Moreover, there is no reaction between the CO2 and the salt. However, creep processes do not make evaporites great seals for long-term storage (e.g., Bachu, 2000; Lokhorst and Wildenborg, 2005). In terms of available storage space, fractures provide the essential pore space within the lithologies where matrix porosity is low (<10%). Therefore, brittle behaviour of rocks is advantageous due to their ability to fracture. Clay-rich shales may be unsuitable in this case because they show plastic behaviour (Figure 6). Fully or partially cemented fractures may often act as proppants and keep the fractures open even in a relatively ductile rock (e.g., Hilgers and Urai, 2005).
Several criteria have to be considered when evaluating the potential of a sedimentary basin for CCS: 1) its tectonic setting and geology as zones of plate convergence may pose issues of integrity and safety of disposal operations and storage; 2) the basin geothermal regime to determine the potential spatial distribution of various CO2 phases, 3) the hydrodynamic regime of formation waters as CO2 injection in over-pressured aquifers may raise technological and safety issues, 4) economic aspects relating to access and infrastructure, and 5) socio-political conditions that would not restrict the CCS operations (Bachu, 2000). Due to the possibility of CO2 leakage (see CO2 Leakage From Injected Reservoir to Surroundings section), the current focus is mostly placed on offshore sites: re-use of depleted oil and gas fields or closed saline aquifers in the offshore (e.g., K43, 2016). A drawback of oil and gas fields is that most of them are at a considerable distance from the CO2 emitting power plants as in the case of the North Sea region (Lokhorst and Wildenborg, 2005).
Nuclear Waste Disposal
Each lithology has its advantages and disadvantages for nuclear waste disposal (Table 5). The biggest risk of disposal in salt rock is its high solubility (e.g. Hansen and Leigh, 2011). The biggest problem with disposal in shale is that due to its weak nature, disposal facility will leave a much bigger footprint than that in a hard rock. It may also be more difficult to retrieve the containers if something goes wrong—same with salt. Disadvantages of granite is its brittle deformational behaviour, and hence fractures pose the biggest concern for stability and leakage of radionuclides (Figure 5). Some of these risks may be mitigated. For instance, choosing a reservoir in a stable platform with low risk of seismic activity may reduce the risk of faulting and fracturing, and hence connection and leakage of radionuclides into the groundwater. Choosing locations in arid climates or far from the circulation of subsurface/phreatic waters may help reduce the risk of dissolution in the evaporite deposits. Despite the aforementioned risks, the three lithologies provide very good repositories for nuclear waste. Salt is practically impermeable and its high susceptibility for creeping poses a low risk of brittle deformation (Figure 7). Therefore, salt provides mechanically stable environment. Clay-rich shale has high sorption behaviour and very low permeability. Moreover, high porosity, clay-rich shale deforms in a ductile manner, forming a natural barrier around the GDF (Figure 6). Granite is an attractive host rock for nuclear waste disposal for its very low permeability and high strength, and hence high mechanical stability. However, engineered barriers are still needed to seal the space around the containers where tunnelling may have caused damage around the disposal facility (Figure 5; e.g., Lanyon et al., 2009; Tsang et al., 2012; Groff et al., 2016). Having some permeable fractures in granite may be an advantage because it stops high gas pressures developing and creating larger fractures than those that are naturally pressured. Shales have such low permeability and high threshold pressures that in the case of high gas pressures developing, shales may fracture. 3-D full-waveform inversion (FWI) of seismic data can be used to map changes in physical properties caused by the construction of the site, like was done by Bentham et al. (2018) for tunnel-induced fracturing in granite.
Geosciences’ Role in Decarbonisation: Challenges and Opportunities
Technology-Specific Knowledge Gaps
For geothermal heat and energy extraction, predicting the amount of scaling and hence its long-term sustainability remains difficult and not accurate. The dependence of geothermal energy and heat storage technologies on the continued permeability of the subsurface poses a major challenge. We still lack the fundamental knowledge to enable us to predict confidently the timescales of permeability increase or decrease with time as well as associated strategies of mitigating potential problems. Subsurface fracture networks can have a range of attributes (including being absent, or being highly spatially clustered) that could have a big influence on post-stimulation permeably or heat exchange capacity. Moreover, seismicity related to hydrofracturing is still one of the biggest uncertainties for the utilization of deep geothermal reservoirs, especially related to the reactivation of pre-existing faults: we still don’t know whether it is safe to drill near faults (using the advantage of pre-existing fracture systems), or whether it is best to avoid the fault zones (we need to understand the safe drilling conditions). Detailed distribution of pre-existing faults is the most important factor for creating a hazard model before exploiting possibility of a hydraulic fracturing of a reservoir. However, mapping critically stressed faults is limited by the resolution of seismic surveys, and even a 3D seismic survey will not always detect all faults of a size that are relevant for the seismic hazard (Baisch et al., 2016).
The link between temperature and hydrothermal convection along fault zones is more complicated than previously thought. Along the same fault zone the situation can change at short distances as was shown in the unsuccessful Trebur GT1 well in Germany, where major differences in hydraulics existed along strike of the fault with hydraulic convection in the northern part and missing convection in the southern part (Reinecker et al., 2019). Currently there is no method to accurately map the hydrothermal convection along fault zones during the feasibility screening of reservoirs. Seismic methods alone are insufficient to map alterations. Electromagnetic methods (EM) aiming at mapping Earth resistivity may improve the alteration mapping in the case of low-enthalpy resources. Adding structural and stratigraphic information may help overcome the low-resolution problem that arises due to the diffuse nature of the EM wave propagation in the subsurface (Reinecker et al., 2019).
For the heat storage systems, thermal efficiency in different geological conditions is still not fully explored, as well as thermal impact on surrounding areas with drinking water interests (e.g., Kallesøe and Vangkilde-Pedersen, 2019). Here more research is needed on (a) field studies and numerical modelling taking a large variety of interlinked processes into account, and (b) water treatment, such as the use of CO2 as a treatment agent.
For the CCS systems, further investigation is needed to understand three-phase (water, gaseous CO2 and liquid CO2) relative permeability and hysteresis. Such three-phase related changes may result in the formation of CO2 leakage pathways and may lead to a self-limiting feedback that decreases the leakage rate (e.g., Pruess, 2005). However, the capillary properties of three-phase flow are not well-known and can therefore be predicted with very high uncertainty. Moreover, the geomechanical implications of CO2 leakage related to cooling effects, especially when liquid CO2 is formed, have not been investigated yet to our knowledge. Predicting top seal capacity of saline aquifers is still challenging (e.g. Lokhorst and Wildenborg, 2005).
Lithology-Specific Knowledge Gaps
Carbonates
Further work is needed on the interaction of fault and fracture systems in carbonates; we should be able to predict, what the conditions are, at which the faults are cut by fractures. Complex hydromechanical behaviour of fluid-induced fractures, including their geometry and interaction with pre-existing fractures, has not yet been completely understood (e.g., Kaminskaite, 2019). Often the flow of organic acidic, high-temperature formation waters, and Mg-rich corrosive fluids along fractures and faults form productive reservoirs at depths of up to 7 km (e.g., Zhu et al., 2010; Biehl et al., 2016; Menezes et al., 2019; Jiu et al., 2020; Ukar et al., 2020). How to predict when those fractures will stay open or closed in deep carbonates? Moreover, predicting deep high permeability flow paths poses a continuing challenge. In particular, it remains unclear if matrix permeability is, in the long-term, more important than fracture network permeability as fracture permeability may be in fact only intermittently important. Mathematical and computational frameworks remain a challenge in capturing fault and fracture opening, closing or failure across time and spatial scales with THMC feedback mechanisms that affect mechanical stability (Pyrak-Nolte et al., 2015).
Sandstone
Most sandstones are not pure quartzose sandstones, and even small impurities internally or as layers not seismically resolvable may have a large influence on the precipitation and dissolution behaviour, as, for instance, sandstones at Zion national park, Utah, or Pembroke, where chemical reactions are strongly localized and can be distinguished by colour change and oxidation states. However, experiments are generally done on pure sandstones, thus reservoir characterisation becomes difficult when impure sandstone reservoirs are in question.
Balancing production capacity with sufficient injection capacity remains the biggest utilisation challenge in geothermal, especially low temperature reservoirs. Tracing the chemical changes and history matching in the production/injection brines is critical. This may result in impaired system performance and unusual exotic scaling, as, for instance, native lead precipitation in Rotliegend reservoirs of Netherlands.
Granite
A lot of research has been done on fractures in granitic rocks, including observations from deep cores and spatial arrangement (Wang X. et al., 2019). However, more needs to be learned about specific attributes like length distribution, spatial arrangement, and porosity structure. Moreover, there is a wealth of information from the oil and gas industry on hydraulic simulation of tight sandstone, carbonate and shale reservoirs, but not much is known about injecting water into granites/basalt etc. on a field scale and chemical/physical reactions associated with it on a long timescale.
Shales
Linking up the scales is particularly difficult for shales. For instance, upscaling fracture toughness would require measuring the anisotropy and other heterogeneities in elastic parameters and interfacial surface energy from nano- up to macro-meter scale, and developing micromechanical models in which several scales can be linked (Ilgen et al., 2017, and references therein). Fracture systems in shales show dynamic behaviour: they can change their producibility, rock strength and the propensity to interact with hydraulic-fracture stimulation (Gale et al., 2014). Therefore, it is challenging to predict how long the fractures in shales stay open for. What are their properties over long geological timescales? Will the fractures close and is there anything that can be done to enhance closure (i.e., change water chemistry)? In weak shales, fully cemented fractures have a capacity to widen due to the force of crystallization (Hilgers and Urai, 2005). Crystallization force could also contribute to fracture development along the cement-fracture wall interface, however, the load exerted by growing crystals is poorly understood (Laubach et al., 2019) and hence the effect of crystallization on fracture opening cannot be simulated yet.
Evaporites
Even though there has been over 20 years of intense research on the permeability and flow behaviour of salt rocks (e.g., Beauheim and Roberts, 2000; Hovland et al., 2008; Smith et al., 2013; Bertoni and Cartwright, 2015; Ghanbarzadeh et al., 2015; Rizzo et al., 2020), many uncertainties still exist in this field. Does salt become permeable at greater depth? Does it become permeable in the event of vessel failure? In particular, the changes in salt properties over long timescales remains elusive.
Coal
Due to the long heritage of coal mining, there is a large variety of coal mine types in the world, such as bell-pits, room-and-pillar and long-wall workings (e.g., Lake et al., 1992; Spearing, 1994). Owing to the different techniques employed for their exploitation, they all have different layouts, and hence mechanical integrity and pore space. For instance, an area mined by room-and-pillar methods can be assumed to have around 50% of the original void space remaining and, for long-wall mining, around 20% of the original void space remains open/not collapsed (Younger and Adams, 1999). Research on geothermal potential of coal mine water has greatly increased in the past few years (e.g., Hall et al., 2011; Athresh et al., 2015; Athresh et al., 2016; Loredo et al., 2017; Banks et al., 2019), but such old workings still remain largely unexplored. Modelling of geomechanical state of flooded workings along with ground-proofing results is urgently needed for different types of coal mines, especially taking into account the cyclical heat loading caused by fluid injection and extraction during the heat storage and geothermal coal mine water utilization (e.g., Todd et al., 2019). Also, very little work has been done so far to assess whether cycling of injection of different gases could increase methane desorption and increase CO2 adsorption in the CCS systems.
The Biggest Uncertainties in all Decarbonising Applications
Among the most important and challenging problems in all decarbonisation technologies is identifying and understanding key influences on fracture pattern development and how to recognize these influences with the limited samples, sparse subsurface and ambiguous outcrop observations that are typically available. Laubach et al. (2019) proposed that in diagenetic settings chemical reactions within rocks have a profound influence on the development of natural fracture systems, and their role in fracture pattern development has not been systematically explored. The extent and texture of cements, fluid inclusions and other features can tie fractures to the processes that formed them while also constraining fracture timing and rates, more studies are thus needed to find the relationships between the diagenetic events and fracture pattern development (Laubach et al., 2019).
Traditionally, chemical reactions have been viewed as slow and acting over geological timescales, however, whenever a mineral comes into contact with a fluid with which it is out of equilibrium, the system will try to equilibrate and hence the chemical reactions will occur (e.g., Putnis et al., 2009). Therefore, in the realm decarbonisation technologies are implemented, where fluid or gas is often cyclically injected, fluid-rock interactions will be instant and chemical reactions will occur in short time scales as the system will try to reach equilibrium after each injection, resulting in dissolution-precipitation processes (e.g., Vaitiekūnas, 2012). Not many studies have been reported on the observations from existing demonstration or commercial plants in terms of chemical reactions within the reservoirs, therefore predicting the timescales and extent of reservoir clogging or dissolution is difficult.
Even though reservoir characteristics and THMC processes occurring within these reservoirs depend on a large number of parameters (Table 2), models vary in complexity depending on data availability and study objectives. Comprehensive simulation softwares and codes of all THMC processes simulated in a single evaluation model are still lacking (e.g., Jacquey et al., 2016).
Closing Knowledge Gaps
The dynamic nature of feedback mechanisms, locally and regionally, make comprehensive and accurate modelling very difficult. Long-term sustainability can only be accurately predicted by closing the aforementioned and other knowledge gaps associated with the rates of changes of the physiochemical properties of subsurface strata at various conditions. That can be done in several ways, including:
(1) Knowledge transfer from fossil fuel industry and sharing of data publicly (e.g., Erdlac, 2006; Bu et al., 2012; Groff et al., 2016): This should include the re-skilling and repurposing/deployment of highly skilled and experienced oil and gas professionals, especially engineers and geologists;
(2) Knowledge transfer from active decarbonisation plants around the world to allow optimization and sustainable implementation of technologies in other countries: Examples include storing CO2 in basalt in the CarbFix Pilot Project in Iceland (Matter et al., 2009), geothermal energy plants such as Reykjanes, Krafla (Friðleifsson et al., 2015; Friðleifsson et al., 2019) or Larderello, Italy (Batini et al., 2003), and ATES at Eindhoven University of Technology in Netherlands (Kallesøe and Vangkilde-Pedersen, 2019);
(3) Short and long term laboratory experiments: For instance, scaling experiments (e.g., Stáhl et al., 2000), porosity-permeability measurements on fault rocks (e.g., Michie et al., 2020a; Michie et al., 2020b) coupled with in-depth microstructural studies (e.g., Kaminskaite et al., 2019; Kaminskaite et al., 2020);
(4) Experiments at test sites, such as the UKGEOS coal mine geothermal test site in Glasgow, nuclear waste disposal sites in Olkiluoto, Finland (Siren, 2015), SKB in Sweden (Rosborg and Werme, 2008), Mont-Terri in Switzerland (Tsang et al., 2012), Mol-Dessel in Belgium (Desbois et al., 2010), and Bure and Tournemire in France (Armand et al., 2007; Matray et al., 2007).
(5) Study of natural geological systems for long term behaviour and comparisons of predictions based on laboratory experiments coupled with numerical simulations: For instance, outcrops and/or core plugs taken out from natural geothermal systems where hydrothermal fluids have been flowing over long timescales (>102–104 yrs) or fossil geothermal systems provide us with the examples of how hydrothermal fluids have affected the rocks on a large scale and how long the system has sustained the flow for (e.g., Major et al., 2018);
(6) Numerical modelling using sophisticated and continuously improving codes, e.g.,: Microstructural modelling using hybrid approaches e.g., ELLE (Vass et al., 2014; Piazolo et al., 2019; Koehn et al., 2020) or codes for coupled THMC processes in porous and fractured media such as OpenGeoSys (e.g., Jacquey et al., 2016; Todd et al., 2019) and TOUGH-FLAC that links the multiphase flow and heat transport simulator TOUGH2 with the geomechanical simulator FLAC3D and a recently improved version of the EWASG Equation-Of-State (EOS) module of TOUGH2 that includes the thermodynamic properties of aqueous fluids of variable salinity (e.g., Blanco-Martín et al., 2018).
Combination of these approaches are especially important as each approach has its limitations. For example, project combining field observations to evaluate long-term effects, laboratory experiments to quantify these effects and their products, and high-end, multi-scale modelling so that quantitative evaluation would be possible are powerful and necessary to evaluate the sustainability of a resource. We need to go from micro to macro scale because the main questions can only be answered with microstructural work, linking structure and physical processes to chemical/biological processes as well as their interaction, looking at this in a dynamic sense rather than static.
Interdisciplinary Research—A Necessity
Specific areas of the subsurface strata may have more than one possible function (e.g., storage vs. heat/energy generation) and the potential to be used for more than one energy type (e.g., compressed air vs. hydrogen), thus it is important to consider the best use of the given subsurface structures and reservoirs. Geoscience research can thus present national, regional and local authorities with opportunities for low carbon economic regeneration. However, an integrated, interdisciplinary collaboration linking geoscience with social science, end-users and stakeholders is crucial to carry out these opportunities (e.g., Bush and Bale, 2019; Rattle et al., 2020). Determining the optimal integration of solutions requires balancing numerous actors and places with a number of technologies while taking into account the material properties of the subsurface. The “real” life cycle footprint should always be considered and evaluated as well as realistic cost benefit analysis which requires incorporation of stakeholders throughout, from sponsor to supporters. The role of public engagement should not be overlooked, as working with communities to develop their local geoassets can attract investment opportunities, whereas their opposition would be a big stopper (e.g., Kowalewski et al., 2014; Kluge and Ziefle, 2016). Executing pilots will demonstrate competence in securing funding and gaining public stakeholder acceptance.
Conclusion
Installation of energy transition technologies using the subsurface will result in thermal, hydrological, mechanical and chemical perturbations within the subsurface, especially where fluid or gas is cyclically injected as fluid-rock interactions will be instant and chemical reactions will occur in short time scales as the system will try to reach equilibrium after each injection, resulting in dissolution-precipitation processes. Therefore, understanding the imposed effects and consequent dynamics within the system are crucial during the feasibility screening. The nature of these perturbations varies in different lithologies and with respect to different technologies. For instance, swelling of clays and coal is a good property considering sealing capacity of rocks, whereas it is an unfavourable characteristic for a CCS and geothermal reservoir rock. Brittle deformation behaviour of crystalline rocks may be a desired quality for the CCS and geothermal reservoirs, but a poor property for the GDF. Moreover, each site has unique characteristics and long term performance assessment involves a thorough characterization of each site, the identification of processes of mass/heat transfer and transport, and, finally, the modelling of the overall evolution of the decarbonising systems.
In particular, the following key knowledge gaps need to be urgently addressed to allow for reliable assessment of the suitability of a particular site for geothermal heat and energy extraction, UTES, CCS and nuclear waste disposal technology implementation and operation:
(1) Identification and in-depth understanding of key influences on fracture pattern development and their link to chemical reaction rates. The extent and timescales of chemical reactions remain poorly understood.
(2) Accurate prediction of the sustainability and longevity of the geothermal systems as well performance and reservoir permeability can be significantly reduced by mineral precipitation during production. We still lack quantitative tools for their assessment.
(3) Reliable assessment of long term integrity of the seals necessary for safe CO2 storage. In-depth knowledge of the geomechanical implications and risks associated with CO2 injection in terms of increased pore pressure, thermal cracking and/or mineral reactions is still largely lacking. Similarly, predicting top seal capacity of saline aquifers remain challenging.
(4) Data availability necessary to determine and continuously monitor locations for nuclear waste storage. Locations that are mechanically, physically and chemically stable are needed to reduce risk of circulation of subsurface/phreatic waters. However, multi-scale, time resolved data is still largely lacking.
Understanding of seismic risks of energy transition technology implementation and operation. For example, for low-permeability reservoirs used for geothermal energy extraction, hydrofracturing-induced seismicity still poses one of the biggest public concerns. In this case, it is still not resolved if it is safe to drill near faults using the advantage of pre-existing fracture systems, or whether it is best to avoid the fault zones. A particular challenge in all aspects of research on subsurface strata is to link rock characteristics across large time and length scales. We need to combine research from large scale to microscale to link the structures and physical processes to chemical/biological processes as well as their interaction in a dynamic sense to realize how the system behaves on long timescales. High-end modelling coupling all thermal, hydrological, mechanical and chemical processes and their dynamics and interactions into a single code is very important for more accurate reservoir performance and hazard assessment.
Subsurface opportunities recognised by the geoscientists in geoenergy transition can only be realized with the help of an interdisciplinary collaboration between geoscience, social science, end-users and stakeholders, all of them working in accordance. Geoscientists can identify the opportunities and reduce uncertainty in order to minimise the risks associated with the decarbonisation applications; social scientists can recognise and clear out the concerns risen by the posed risks; and end-users and stakeholders can make the right decisions for the decarbonisation opportunities to the realized. Everyone has a crucial part to play.
Author Contributions
IK—literature review, discussion, and review. SP—discussion and review. AE—discussion and review. NS—discussion and review. QF—discussion and review.
Funding
This work has been funded through the Strategic Priorities Fund provided by Research England as part of the Sustainable Geoenergy Solutions programme at the University of Leeds.
Conflict of Interest
Authors IK and QF were employed by the company Petriva Ltd.
The remaining authors declare that the research was conducted in the absence of any commercial or financial relationships that could be construed as a potential conflict of interest.
Publisher’s Note
All claims expressed in this article are solely those of the authors and do not necessarily represent those of their affiliated organizations, or those of the publisher, the editors and the reviewers. Any product that may be evaluated in this article, or claim that may be made by its manufacturer, is not guaranteed or endorsed by the publisher.
Acknowledgments
The authors would like to thank the other interdisciplinary team members for their invaluable discussions during the project: Rachael Spraggs, James Van Alstine, Eric Peterson, David Hodgson, Natasha Barlow, Anne Velenturf, Imogen Rattle and Amir Mohtaj Khorasani. Further we are grateful to two reviewers and a handling associate editor Christopher Yeomans for a critical review and useful discussions on the content of this paper.
References
Amaya, A., Scherer, J., Muir, J., Patel, M., and Higgins, B. (2020). “GreenFire Energy Closed-Loop Geothermal Demonstration Using Supercritical Carbon Dioxide as Working Fluid,” in PROCEEDINGS, 45th Workshop on Geothermal Reservoir Engineering. Stanford University, Stanford, California, February 10-12, 2020.
Aminu, M. D., Nabavi, S. A., Rochelle, C. A., and Manovic, V. (2017). A Review of Developments in Carbon Dioxide Storage. Appl. Energy 208, 1389–1419. doi:10.1016/j.apenergy.2017.09.015
Anderson, R. L., Ratcliffe, I., Greenwell, H. C., Williams, P. A., Cliffe, S., and Coveney, P. V. (2010). Clay Swelling—A Challenge in the Oilfield. Earth-Science Rev. 98 (3-4), 201–216. doi:10.1016/j.earscirev.2009.11.003
Andre, B. J., and Rajaram, H. (2005). Dissolution of Limestone Fractures by Cooling Waters: Early Development of Hypogene Karst Systems. Water Resour. Res. 41, W01015. doi:10.1029/2004wr003331
Ardelan, M. V., and Steinnes, E. (2010). Changes in Mobility and Solubility of the Redox Sensitive Metals Fe, Mn and Co at the Seawater-Sediment Interface Following CO<sub>2</sub> Seepage. Biogeosciences 7, 569–583. doi:10.5194/bg-7-569-2010
Armand, G., Wileveau, Y., Morel, J., Cruchaudet, M., and Rebours, H. (2007). “August. Excavation Damaged Zone (EDZ) in the Meuse/Haute-Marne Underground Research Laboratory,” in Proceedings of the 11th Congress of the International Society for Rock Mechanics (IRSM 2007), Lisbon, Portugal, 9-13 July 2007, 33–36.
Arnórsson, S. (1989). Deposition of Calcium Carbonate Minerals from Geothermal Waters—Theoretical Considerations. Geothermics 18 (1-2), 33–39. doi:10.1016/0375-6505(89)90007-2
Athresh, A. P., Al-Habaibeh, A., and Parker, K. (2015). Innovative Approach for Heating of Buildings Using Water from a Flooded Coal Mine through an Open Loop Based Single Shaft GSHP System. Energy Procedia 75, 1221–1228. doi:10.1016/j.egypro.2015.07.162
Athresh, A. P., Al-Habaibeh, A., and Parker, K. (2016). The Design and Evaluation of an Open Loop Ground Source Heat Pump Operating in an Ochre-Rich Coal Mine Water Environment. Int. J. Coal Geol. 164, 69–76. doi:10.1016/j.coal.2016.04.015
Atkinson, B. K. (1984). Subcritical Crack Growth in Geological materialsJournal of Geophysical Research: Solid Earth. J. Geophys. Res. 89, 4077–4114. doi:10.1029/jb089ib06p04077
Bachu, S. (2000). Sequestration of CO2 in Geological Media: Criteria and Approach for Site Selection in Response to Climate Change. Energy Convers. Manag. 41 (9), 953–970. doi:10.1016/s0196-8904(99)00149-1
Baer, J., and Rigby, J. (1978). Geology of the Crystal Geyser and the Environmental Implications of its Effluent. Grand County, Utah: Utah Geol.
Bailey, M. T., Gandy, C. J., Watson, I. A., Wyatt, L. M., and Jarvis, A. P. (2016). Heat Recovery Potential of Mine Water Treatment Systems in Great Britain. Int. J. Coal Geol. 164, 77–84. doi:10.1016/j.coal.2016.03.007
Baisch, S., Koch, C., Stang, H., Pittens, B., Drijver, B., and Buik, N., 2016. Defining the Framework for Seismic Hazard Assessment in Geothermal Projects V0. Technical Report.
Balat, H., and Öz, C. (2007). Technical and Economic Aspects of Carbon Capture an Storage - A Review. Energy Explor. exploitation 25 (5), 357–392. doi:10.1260/014459807783528883
Banks, D., Athresh, A., Al-Habaibeh, A., and Burnside, N. (2019). Water from Abandoned Mines as a Heat Source: Practical Experiences of Open- and Closed-Loop Strategies, United Kingdom. Sustain. Water Resour. Manag. 5 (1), 29–50. doi:10.1007/s40899-017-0094-7
Banks, D., Younger, P. L., Arnesen, R.-T., Iversen, E. R., and Banks, S. B. (1997). Mine-water Chemistry: the Good, the Bad and the Ugly. Environ. Geol. 32 (3), 157–174. doi:10.1007/s002540050204
Bateman, K., Coombs, P., Noy, D. J., Pearce, J. M., Wetton, P., Haworth, A., et al. (1999). Experimental Simulation of the Alkaline Disturbed Zone Around a Cementitious Radioactive Waste Repository: Numerical Modelling and Column Experiments. Geol. Soc. Lond. Spec. Publ. 157 (1), 183–194. doi:10.1144/gsl.sp.1999.157.01.14
Batini, F., Brogi, A., Lazzarotto, A., Liotta, D., and Pandeli, E. (2003). Geological Features of Larderello-Travale and Mt. Amiata Geothermal Areas (Southern Tuscany, Italy). Episodes 26 (3), 239–244. doi:10.18814/epiiugs/2003/v26i3/015
Baxter, P. J., Baubron, J. C., and Coutinho, R. (1999). Health Hazards and Disaster Potential of Ground Gas Emissions at Furnas Volcano, Sao Miguel, Azores. J. Volcanol. Geotherm. Res. (1–2), 95–106. doi:10.1016/s0377-0273(99)00070-0
Beauheim, R. L., and Roberts, R. M. (2000). Hydrology and Hydraulic Properties of a Bedded Evaporite Formation. J. Hydrology 259 (1-4), 66–88.
Benbow, S. J., Rivett, M. O., Chittenden, N., Herbert, A. W., Watson, S., Williams, S. J., et al. (2014). Potential Migration of Buoyant LNAPL from Intermediate Level Waste (ILW) Emplaced in a Geological Disposal Facility (GDF) for UK Radioactive Waste. J. Contam. hydrology 167, 1–22. doi:10.1016/j.jconhyd.2014.07.011
Bentham, H. L. M., Morgan, J. V., and Angus, D. A. (2018). Investigating the Use of 3-D Full-Waveform Inversion to Characterize the Host Rock at a Geological Disposal Site. Geophys. J. Int. 215 (3), 2035–2046. doi:10.1093/gji/ggy386
Bertoni, C., and Cartwright, J. (2015). Messinian Evaporites and Fluid Flow. Mar. Petroleum Geol. 66, 165–176. doi:10.1016/j.marpetgeo.2015.02.003
Biehl, B. C., Reuning, L., Schoenherr, J., Lüders, V., and Kukla, P. A. (2016). Impacts of Hydrothermal Dolomitization and Thermochemical Sulfate Reduction on Secondary Porosity Creation in Deeply Buried Carbonates: A Case Study from the Lower Saxony Basin, Northwest Germany. AAPG Bull. 100 (4), 597–621. doi:10.1306/01141615055
Biot, M. A. (1956). General Solutions of the Equations of Elasticity and Consolidation for a Porous Material. J. Appl. Mech. 23 (1), 91–96. doi:10.1115/1.4011213
Bjørkum, P. A., Oelkers, E. H., Nadeau, P. H., Walderhaug, O., and Murphy, W. M. (1998). Porosity Prediction in Quartzose Sandstones as a Function of Time, Temperature, Depth, Stylolite Frequency, and Hydrocarbon Saturation. AAPG Bull. 82 (4), 637–648. doi:10.1306/1d9bc5cf-172d-11d7-8645000102c1865d
Blanco Martín, L., Rutqvist, J., and Birkholzer, J. T. (2015). Long-term Modeling of the Thermal-Hydraulic-Mechanical Response of a Generic Salt Repository for Heat-Generating Nuclear Waste. Eng. Geol. 193, 198–211. doi:10.1016/j.enggeo.2015.04.014
Blanco-Martín, L., Rutqvist, J., Battistelli, A., and Birkholzer, J. T. (2018). Coupled Processes Modeling in Rock Salt and Crushed Salt Including Halite Solubility Constraints: Application to Disposal of Heat-Generating Nuclear Waste. Transp. Porous Med. 124 (1), 159–182. doi:10.1007/s11242-018-1057-7
Borojević Šostarić, S., and Neubauer, F. (2012). Principle Rock Types for Radioactive Waste Repositories. Rudarsko-geološko-naftni Zb. 24 (1), 11–18. doi:10.5455/msm.2012.24.274-276
Bossart, P., Trick, T., Meier, P. M., and Mayor, J. C. (2004). Structural and Hydrogeological Characterisation of the Excavation-Disturbed Zone in the Opalinus Clay (Mont Terri Project, Switzerland). Appl. clay Sci. 26 (1-4), 429–448. doi:10.1016/j.clay.2003.12.018
Bourret, S., Stauffer, P., Otto, S., Weaver, D., Johnson, P. J., and Boukalfa, H. (2017). High Level Waste in Salt Repositories: Experiments and Simulations of Evaporation in the Underground-17167. Washington, D.C.: U.S. Department of Energy.
Bowker, K. A., and Shuler, P. J. (1991). Carbon Dioxide Injection and Resultant Alteration of the Weber Sandstone, Rangely Field, Colorado. Am. Assoc. Pet. Geol. Bull. 75, 1489–1499. doi:10.1306/0c9b2975-1710-11d7-8645000102c1865d
Brace, W. F., Silver, E., Hadley, K., and Goetze, C. (1972). Cracks and Pores: a Closer Look. Science 178 (4057), 162–164. doi:10.1126/science.178.4057.162
Brantut, N., Heap, M. J., Meredith, P. G., and Baud, P. (2013). Time-dependent Cracking and Brittle Creep in Crustal Rocks: A Review. J. Struct. Geol. 52, 17–43. doi:10.1016/j.jsg.2013.03.007
Breede, K., Dzebisashvili, K., Liu, X., and Falcone, G. (2013). A Systematic Review of Enhanced (Or Engineered) Geothermal Systems: Past, Present and Future. Geotherm. Energy 1, 1–27. doi:10.1186/2195-9706-1-4
Brehme, M., Blöcher, G., Regenspurg, S., Milsch, H., Petrauskas, S., Valickas, R., et al. (2017). “Approach to Develop a Soft Stimulation Concept to Overcome Formation Damage–A Case Study at Klaipeda, Lithuania,” in Proceedings, 42nd Workshop on Geothermal Reservoir Engineering, Stanford, California, USA, 13 - 15 February 2017, 1–5.
Brehme, M., Nowak, K., Banks, D., Petrauskas, S., Valickas, R., Bauer, K., et al. (2019). A Review of the Hydrochemistry of a Deep Sedimentary Aquifer and its Consequences for Geothermal Operation. Klaipeda, Lithuania: Geofluids.
Brehme, M., Regenspurg, S., Leary, P., Bulut, F., Milsch, H., Petrauskas, S., et al. (2018). Injection-triggered Occlusion of Flow Pathways in Geothermal Operations. Klaipeda, Lithuania: Geofluids.
Brogi, A., Capezzuoli, E., Alçiçek, M. C., and Gandin, A. (2014). Evolution of a Fault-Controlled Fissure-Ridge Type Travertine Deposit in the Western Anatolia Extensional Province: the Çukurbağ Fissure-Ridge (Pamukkale, Turkey). J. Geol. Soc. 171 (3), 425–441. doi:10.1144/jgs2013-034
Brown, A. (1997). Porosity Variation in Carbonates as a Function of Depth. Williston Basin: Mississippian Madison Group.
Brown, D. W. (2000). “January. A Hot Dry Rock Geothermal Energy Concept Utilizing Supercritical CO2 Instead of Water,” in Proceedings of the twenty-fifth workshop on geothermal reservoir engineering, Stanford University, Stanford, California, January 24-26, 2000, 39–50.221.
Bu, X., Ma, W., and Li, H. (2012). Geothermal Energy Production Utilizing Abandoned Oil and Gas Wells. Renew. energy 41, 80–85. doi:10.1016/j.renene.2011.10.009
Buijze, L., van Bijsterveldt, L., Cremer, H., Paap, B., Veldkamp, H., Wassing, B. B., et al. (2019). Review of Induced Seismicity in Geothermal Systems Worldwide and Implications for Geothermal Systems in the Netherlands. Neth. J. Geosciences 98. doi:10.1017/njg.2019.6
Bullock, L. A., Parnell, J., Perez, M., and Armstrong, J. G. T. (2019). Coal Mining-Derived Ochres in the UK: a Potential Selenium Trap. Geol. Today 35 (4), 140–145. doi:10.1111/gto.12279
Burnside, N. M., and Naylor, M. (2014). Review and Implications of Relative Permeability of CO2/brine Systems and Residual Trapping of CO2. Int. J. Greenh. gas control 23, 1–11. doi:10.1016/j.ijggc.2014.01.013
Burton, E. A., and Walter, L. M. (1987). Relative Precipitation Rates of Aragonite and Mg Calcite from Seawater: Temperature or Carbonate Ion Control? Geol 15 (2), 111–114. doi:10.1130/0091-7613(1987)15<111:rproaa>2.0.co;2
Busch, A., Amann, A., Bertier, P., Waschbusch, M., and Krooss, B. M. (2010). “The Significance of Caprock Sealing Integrity for CO2 Storage,” in SPE International Conference on CO2 Capture, Storage, and Utilization, New Orleans, Louisiana, November 2010. doi:10.2118/139588-ms
Busch, A., and Gensterblum, Y. (2011). CBM and CO2-ECBM Related Sorption Processes in Coal: a Review. Int. J. Coal Geol. 87 (2), 49–71. doi:10.1016/j.coal.2011.04.011
Bush, R. E., and Bale, C. S. E. (2019). Energy Planning Tools for Low Carbon Transitions: an Example of a Multicriteria Spatial Planning Tool for District Heating. J. Environ. Plan. Manag. 62 (12), 2186–2209. doi:10.1080/09640568.2018.1536605
Casanova, J., Bodénan, F., Négrel, P., and Azaroual, M. (1999). Microbial Control on the Precipitation of Modern Ferrihydrite and Carbonate Deposits from the Cézallier Hydrothermal Springs (Massif Central, France). Sediment. Geol. 126 (1-4), 125–145. doi:10.1016/s0037-0738(99)00036-6
Chen, B., Wang, F., Shi, J., Chen, F., and Shi, H. (2019). Origin and Sources of Minerals and Their Impact on the Hydrocarbon Reservoir Quality of the PaleogeneLulehe Formation in the Eboliang Area, Northern Qaidam Basin, China. Minerals 9 (7), 436. doi:10.3390/min9070436
Chen, X., Eichhubl, P., Olson, J. E., and Dewers, T. A. (2019). Effect of Water on Fracture Mechanical Properties of Shales. J. Geophys. Res. Solid Earth 124 (3), 2428–2444. doi:10.1029/2018jb016479
Chen, X., Eichhubl, P., Olson, J. E., and Dewers, T. A. (2020). Salinity, pH, and Temperature Controls on Fracture Mechanical Properties of Three Shales and Their Implications for Fracture Growth in Chemically Reactive Fluid Environments. Geomechanics Energy Environ. 21, 100140. doi:10.1016/j.gete.2019.100140
Cui, G., Zhang, L., Tan, C., Ren, S., Zhuang, Y., and Enechukwu, C. (2017). Injection of Supercritical CO 2 for Geothermal Exploitation from Sandstone and Carbonate Reservoirs: CO 2 -Water-Rock Interactions and Their Effects. J. CO2 Util. 20, 113–128. doi:10.1016/j.jcou.2017.05.006
De Simone, S., Vilarrasa, V., Carrera, J., Alcolea, A., and Meier, P. (2013). Thermal Coupling May Control Mechanical Stability of Geothermal Reservoirs during Cold Water Injection. Phys. Chem. Earth, Parts A/B/C. 64, 117–126. doi:10.1016/j.pce.2013.01.001
Della Porta, G. (2015). Carbonate Build-Ups in Lacustrine, Hydrothermal and Fluvial Settings: Comparing Depositional Geometry, Fabric Types and Geochemical Signature. Sp 418 (1), 17–68. doi:10.1144/sp418.4
Desbois, G., Urai, J. L., and De Craen, M. (2010). In-situ and Direct Characterization of Porosity in Boom Clay (Mol Site, Belgium) by Using Novel Combination of Ion Beam Cross-Sectioning, SEM and Cryogenic Methods. Motivations, First Results and Perspectives. Belgium: External Report of the Belgian Nuclear Research Centre.
Dideriksen, K., Christiansen, B. C., Frandsen, C., Balic-Zunic, T., Mørup, S., and Stipp, S. L. S. (2010). Paleo-redox Boundaries in Fractured Granite. Geochimica Cosmochimica Acta 74 (10), 2866–2880. doi:10.1016/j.gca.2010.02.022
Diehl, T., Kraft, T., Kissling, E., and Wiemer, S. (2017). The Induced Earthquake Sequence Related to the St. Gallen Deep Geothermal Project (Switzerland): Fault Reactivation and Fluid Interactions Imaged by Microseismicity. J. Geophys. Res. Solid Earth 122 (9), 7272–7290. doi:10.1002/2017jb014473
Donselaar, M. E., Groenenberg, R. M., and Gilding, D. T. (2015). “Reservoir Geology and Geothermal Potential of the Delft Sandstone Member in the West Netherlands Basin,” in Proceedings World Geothermal Congress.
Dou, H. P. (2012). Research of Chemical Clogging during Waste Geothermal Water Re-injection Based on Hydrogeochemical Simulation. M.Sc. Thesis. Xi’an: Chang’an University. (in Chinese with English abstract).
Dove, P. M. (1995). Geochemical Controls on the Kinetics of Quartz Fracture at Subcritical Tensile Stresses. J. Geophys. Res. 100 (B11), 22349–22359. doi:10.1029/95jb02155
Duro, L., Domènech, C., Grivé, M., Roman-Ross, G., Bruno, J., and Källström, K. (2014). Assessment of the Evolution of the Redox Conditions in a Low and Intermediate Level Nuclear Waste Repository (SFR1, Sweden). Appl. Geochem. 49, 192–205. doi:10.1016/j.apgeochem.2014.04.015
Dusseault, M. B. (2004). Coupled Processes and Petroleum Geomechanics. Elsevier Geo- Eng. Book Ser. 2, 49–62. doi:10.1016/S1571-9960(04)80022-3
Ehrenberg, S. N., and Nadeau, P. H. (2005). Sandstone vs. Carbonate Petroleum Reservoirs: A Global Perspective on Porosity-Depth and Porosity-Permeability Relationships. Bulletin 89, 435–445. doi:10.1306/11230404071
Erdlac, R. J. (2006). A Resource Assessment of Geothermal Energy Resources for Converting Deep Gas Wells in Carbonate Strata into Geothermal Extraction Wells: A Permian Basin Evaluation. Odessa, Texas: The University of Texas of the Permian Basin Center for Energy & Economic Diversification. No. DOE/GO/85023.
Erőss, A., Mádl-Szőnyi, J., and Csoma, A. É. (2008). Characteristics of Discharge at Rose and Gellért Hills, Budapest, Hungary. Cent. Eur. Geol. 51 (3), 267–281.
Evans, K. F., Zappone, A., Kraft, T., Deichmann, N., and Moia, F. (2012). A Survey of the Induced Seismic Responses to Fluid Injection in Geothermal and CO2 Reservoirs in Europe. Geothermics 41, 30–54. doi:10.1016/j.geothermics.2011.08.002
Ewing, R. C., Whittleston, R. A., and Yardley, B. W. D. (2016). Geological Disposal of Nuclear Waste: a Primer. Elements 12 (4), 233–237. doi:10.2113/gselements.12.4.233
Feldrappe, H., Obst, K., and Wolfgramm, M. (2007). “Evaluation of Sandstone Aquifers of the North German Basin: a Contribution to the „Geothermal Information System of Germany,” in Proceedings of the European Geothermal Congress, Unterhaching, Germany, 30 May -1 June 2007, 1–8.
Folk, R. L. (1993). SEM Imaging of Bacteria and Nannobacteria in Carbonate Sediments and Rocks. J. Sediment. Res. 63 (5), 990–999. doi:10.1306/d4267c67-2b26-11d7-8648000102c1865d
Friðleifsson, G. Ó., Albertsson, A., Stefánsson, A., Şórólfsson, G., Mesfin, K. G., Sigurğsson, K., et al. (2019). The Reykjanes DEEPEGS Demonstration Well–IDDP-2.
Friðleifsson, G. Ó., Pálsson, B., Albertsson, A., Stefánsson, B., Gunnlaugsson, E., Ketilsson, J., et al. (2015). “Þ.: IDDP-1 Drilled into Magma – World’s First Magma-EGS System Created,” in Proceeding, World Geothermal Congress, Melbourne, Australia, 19-25 April 2015.
Gale, J. F., Elliott, S. J., and Laubach, S. E. (2018). “September. Hydraulic Fractures in Core from Stimulated Reservoirs: Core Fracture Description of HFTS Slant Core, Midland Basin, West Texas,” in Unconventional Resources Technology Conference, Houston, Texas, 23-25 July 2018 (Society of Exploration Geophysicists, American Association of Petroleum Geologists, Society of Petroleum Engineers), 1340–1357.
Gale, J. F. W., Laubach, S. E., Olson, J. E., Eichhuble, P., and Fall, A. (2014). Natural Fractures in Shale: A Review and New Observations. Bulletin 98 (11), 2165–2216. doi:10.1306/08121413151
Garrison, R. E. (1981). Diagenesis of Oceanic Carbonate Sediments: A Review of the DSDP Perspective. McLean, Virginia: GeoScienceWorld.
Gens, A., Olivella, S., and Valleján, B. (2001). Analysis of Gas Phase Transport Phenomena in Compacted Clay Barriers.
Gensterblum, Y., Merkel, A., Busch, A., Krooss, B. M., and Littke, R. (2014). Gas Saturation and CO2 Enhancement Potential of Coalbed Methane Reservoirs as a Function of Depth. Bulletin 98 (2), 395–420. doi:10.1306/07021312128
Genter, A., Baujard, C., Cuenot, N., Dezayes, C., Kohl, T., Masson, F., et al. (2016). “Geology, Geophysics and Geochemistry in the Upper Rhine Graben: the Frame for Geothermal Energy Use,” in European Geothermal Congress, Strasbourg, France, 19-24 Sept 2016.
Genter, A., Traineau, H., Ledésert, B., Bourgine, B., and Gentier, S. (2000). “Over 10 Years of Geological Investigations within the HDR Soultz Project, France,” in World Geothermal Congress, Kyushu-Tohoku, Japan, May 28-June 10, 2000, 3707–3712.
Ghanbarzadeh, S., Hesse, M. A., Prodanovi , M., and Gardner, J. E. (2015). Deformation-assisted Fluid Percolation in Rock Salt. Science 350 (6264), 1069–1072. doi:10.1126/science.aac8747
Gholami, R., Raza, A., Andersen, P., Escalona, A., Cardozo, N., Marín, D., et al. (2021). Long-term Integrity of Shaly Seals in CO2 Geo-Sequestration Sites: An Experimental Study. Int. J. Greenh. Gas Control 109, 103370. doi:10.1016/j.ijggc.2021.103370
Glover, P. W. J., Baud, P., Darot, M., Meredith, P. G., Boon, S. A., LeRavalec, M., et al. (1995). α/β Phase Transition in Quartz Monitored Using Acoustic Emissions. Geophys. J. Int. 120 (3), 775–782. doi:10.1111/j.1365-246x.1995.tb01852.x
Goldscheider, N., Mádl-Szőnyi, J., Erőss, A., and Schill, E. (2010). Review: Thermal Water Resources in Carbonate Rock Aquifers. Hydrogeol. J. 18 (6), 1303–1318. doi:10.1007/s10040-010-0611-3
Grare, A., Lacombe, O., Mercadier, J., Benedicto, A., Guilcher, M., Trave, A., et al. (2018). Fault Zone Evolution and Development of a Structural and Hydrological Barrier: the Quartz Breccia in the Kiggavik Area (Nunavut, Canada) and its Control on Uranium Mineralization. Minerals 8 (8), 319. doi:10.3390/min8080319
Grattoni, C. A., Ahsan, M., Durucan, S., and Jing, X. D. (2016). “Measurements of Petrophysical Properties of Coal for Co2 Sequestration,” in Oral presentation of paper SCA2006-10 given at the International Symposium of the Society of Core Analysts, Trondheim, Norway, 12-16 September, 2006, 12–16.
Green, S., O'Connor, S., Swarbrick, R., and Waters, K. (2016). A Hydrodynamic Model and Associated Spill-point Map for the Huntington Field, UK Central North Sea. Pet. Geol. Conf. Ser. 8, 399–412. doi:10.1144/pgc8.7
Grigoli, F., Cesca, S., Rinaldi, A. P., Manconi, A., López-Comino, J. A., Clinton, J. F., et al. (2018). The November 2017 M W 5.5 Pohang Earthquake: A Possible Case of Induced Seismicity in South Korea. Science 360 (6392), 1003–1006. doi:10.1126/science.aat2010
Grigsby, C. O., Tester, J. W., Trujillo, P. E., Counce, D. A., Abbott, J., Holley, C. E., et al. (1983). Rock-water Interactions in Hot Dry Rock Geothermal Systems: Field Investigations of In Situ Geochemical Behavior. J. Volcanol. Geotherm. Res. 15 (1-3), 101–136. doi:10.1016/0377-0273(83)90097-5
Groff, F., Jefferies, N., and Norris, S. (2016). Development of A UK Approach to Sealing Deep Site Investigation Boreholes: Knowledge Transfer from Other Industries.
Guido, D. M., and Campbell, K. A. (2018). Upper Jurassic Travertine at El Macanudo, Argentine Patagonia: a Fossil Geothermal Field Modified by Hydrothermal Silicification and Acid Overprinting. Geol. Mag. 155 (6), 1394–1412. doi:10.1017/s0016756817000498
Gunter, W. D., Bachu, S., and Benson, S. (2004). The Role of Hydrogeological and Geochemical Trapping in Sedimentary Basins for Secure Geological Storage of Carbon Dioxide. Geol. Soc. Lond. Spec. Publ. 233 (1), 129–145. doi:10.1144/gsl.sp.2004.233.01.09
Guo, X., Li, Y., Liu, R., and Wang, Q. (2014). Characteristics and Controlling Factors of Micropore Structures of the Longmaxi Shale in the Jiaoshiba Area, Sichuan Basin. Nat. Gas. Ind. B 1 (2), 165–171. doi:10.1016/j.ngib.2014.11.007
Hall, A., Scott, J. A., and Shang, H. (2011). Geothermal Energy Recovery from Underground Mines. Renew. Sustain. Energy Rev. 15 (2), 916–924. doi:10.1016/j.rser.2010.11.007
Hall, D. S., Behazin, M., Jeffrey Binns, W., and Keech, P. G. (2021). An Evaluation of Corrosion Processes Affecting Copper-Coated Nuclear Waste Containers in a Deep Geological Repository. Prog. Mater. Sci. 118, 100766. doi:10.1016/j.pmatsci.2020.100766
Hansen, F. D., and Leigh, C. D. (2011). Salt Disposal of Heat-Generating Nuclear Waste. Albuquerque, NM: Sandia National Laboratories, 110.
Headworth, H. G., Puri, S., and Rampling, B. H. (1980). Contamination of a Chalk Aquifer by Mine Drainage at Tilmanstone, East Kent, U.K. Q. J. Eng. Geol. Hydrogeology 13 (2), 105–117. doi:10.1144/gsl.qjeg.1980.013.02.05
Hedin, R. S., Nairn, R. W., and Kleinmann, R. L. (1994). Passive Treatment of Coal Mine Drainage. Washington, D.C: US Department of the Interior, Bureau of Mines.
Heinemann, N., Stewart, R. J., Wilkinson, M., Pickup, G. E., and Haszeldine, R. S. (2016). Hydrodynamics in Subsurface CO 2 Storage: Tilted Contacts and Increased Storage Security. Int. J. Greenh. Gas Control 54, 322–329. doi:10.1016/j.ijggc.2016.10.003
Hepple, R. P., and Benson, S. M. (2005). Geologic Storage of Carbon Dioxide as a Climate Change Mitigation Strategy: Performance Requirements and the Implications of Surface Seepage. Environ. Geol. 47, 576–585. doi:10.1007/s00254-004-1181-2
Heroy, W. B. (1957). The Disposal of Radioactive Waste on Land. Washington, DC: National Academies Press. Appendix F, Disposal of radioactive waste in salt cavities.
Hilgers, C., and Urai, J. L. (2005). On the Arrangement of Solid Inclusions in Fibrous Veins and the Role of the Crack-Seal Mechanism. J. Struct. Geol. 27 (3), 481–494. doi:10.1016/j.jsg.2004.10.012
Hill, B., Hovorka, S., and Melzer, S. (2013). Geologic Carbon Storage through Enhanced Oil Recovery. Energy Procedia 37, 6808–6830. doi:10.1016/j.egypro.2013.06.614
Hovland, M., Fichler, C., Rueslatten, H. G., Odinsen, T., Vindstad, J. E., and Johnsen, H. K. (2008). Origin and Permeability of Salt. Report Number: Integrated Ocean Drilling Program. Sci. Drill. Propos.. doi:10.13140/RG.2.1.5088.7522
Hu, J., Sun, Q., and Pan, X. (2018). Variation of Mechanical Properties of Granite after High-Temperature Treatment. Arab. J. Geosci. 11 (2), 43. doi:10.1007/s12517-018-3395-8
Huang, W.-L., Longo, J. M., and Pevear, D. R. (1993). An Experimentally Derived Kinetic Model for Smectite-To-Illite Conversion and its Use as a Geothermometer. Clays Clay Minerals 41 (2), 162–177. doi:10.1346/ccmn.1993.0410205
Ilgen, A. G., Heath, J. E., Akkutlu, I. Y., Bryndzia, L. T., Cole, D. R., Kharaka, Y. K., et al. (2017). Shales at All Scales: Exploring Coupled Processes in Mudrocks. Earth-Science Rev. 166, 132–152. doi:10.1016/j.earscirev.2016.12.013
IPCC (2013). “Climate Change 2013: The Physical Science Basis,”. Contribution of Working Group I to the Fifth Assessment Report of the Intergovernmental Panel on Climate Change. Editors T. F. Stocker, D. Qin, G. K. Plattner, M. Tignor, S. K. Allen, J. Boschunget al. (Cambridge, United Kingdom: Cambridge University Press), 1535.
Jacquey, A. B., Cacace, M., Blöcher, G., Watanabe, N., Huenges, E., and Scheck-Wenderoth, M. (2016). Thermo-poroelastic Numerical Modelling for Enhanced Geothermal System Performance: Case Study of the Groß Schönebeck Reservoir. Tectonophysics 684, 119–130. doi:10.1016/j.tecto.2015.12.020
Jiu, B., Huang, W., Mu, N., and He, M. (2020). Effect of Hydrothermal Fluids on the Ultra-deep Ordovician Carbonate Rocks in Tarim Basin, China. J. Petroleum Sci. Eng. 194, 107445. doi:10.1016/j.petrol.2020.107445
Johnson, K. S. (2007). Evaporite-karst Problems and Studies in the USA. Environ. Geol. 53 (5), 937–943. doi:10.1007/s00254-007-0716-8
Jones, B., Renaut, R. W., and Rosen, M. R. (2000). Trigonal Dendritic Calcite Crystals Forming from Hot Spring Waters at Waikite, North Island, New Zealand. J. Sediment. Res. 70 (3), 586–603. doi:10.1306/2dc4092a-0e47-11d7-8643000102c1865d
Jones, B. (2017). Review of Calcium Carbonate Polymorph Precipitation in Spring Systems. Sediment. Geol. 353, 64–75. doi:10.1016/j.sedgeo.2017.03.006
K43, (2016). K43: Field Development Report. North Yorkshire, United Kingdom: White Rose Project. 227p.. Technical: Storage. Available at: https://assets.publishing.service.gov.uk/government/uploads/system/uploads/attachment_data/file/531187/K43_Field_Development_Report.pdf.
Kallesøe, A. J., and Vangkilde-Pedersen, T. (2019). Underground Thermal Energy Storage (UTES) – State-Of-The-Art, Example Cases and Lessons Learned. HEATSTORE Project Report, GEOTHERMICA – ERA NET Cofund Geothermal. 130 pp + appendices.
Kaminskaite, I., Fisher, Q. J., and Michie, E. A. H. (2020). Faults in Tight Limestones and Dolostones in San Vito Lo Capo, Sicily, Italy: Internal Architecture and Petrophysical Properties. J. Struct. Geol. 132, 103970. doi:10.1016/j.jsg.2019.103970
Kaminskaite, I., Fisher, Q. J., and Michie, E. A. H. (2019). Microstructure and Petrophysical Properties of Deformation Bands in High Porosity Carbonates. J. Struct. Geol. 119, 61–80. doi:10.1016/j.jsg.2018.12.001
Kaminskaite, I. (2019). Impact of Faults on Fluid Flow in Carbonates. Doctoral dissertation. Leeds, England: University of Leeds.
Karolytė, R., Johnson, G., Yielding, G., and Gilfillan, S. M. V. (2020). Fault Seal Modelling - the Influence of Fluid Properties on Fault Sealing Capacity in Hydrocarbon and CO2 Systems. Pet. Geosci. 26, 481–497. doi:10.1144/petgeo2019-126
Kasztelewicz, A., and Tomaszewska, B. (2019). “The Quality of Geothermal Reservoir of the Lower Jurassic Aquifer in the Mogilno-Łódź Trough (Polish Lowlands),” in E3S Web of Conferences (Les Ulis, France: EDP Sciences), 133, 02004. doi:10.1051/e3sconf/201913302004E3S
Kato, H., Kamei, J., and Kitao, K. (2000). Fluid Flow-Precipitation Model of Anhydrite Scale in Wellbore-Case Study of the Sumikawa Production Well, SC-1. J. Geotherm. Res. Soc. Jpn. 22, 171–185. (In Japanese with English abstract).
Kaven, J. O., Hickman, S. H., McGarr, A. F., and Ellsworth, W. L. (2015). Surface Monitoring of Microseismicity at the Decatur, Illinois, CO2Sequestration Demonstration Site. Seismol. Res. Lett. 86 (4), 1096–1101. doi:10.1785/0220150062
Kele, S., Demény, A., Siklósy, Z., Németh, T., Tóth, M., and Kovács, M. B. (2008). Chemical and Stable Isotope Composition of Recent Hot-Water Travertines and Associated Thermal Waters, from Egerszalók, Hungary: Depositional Facies and Non-equilibrium Fractionation. Sediment. Geol. 211 (3-4), 53–72. doi:10.1016/j.sedgeo.2008.08.004
Khishvand, M., Oraki Kohshour, I., Alizadeh, A. H., Piri, M., and Prasad, S. (2019). A Multi-Scale Experimental Study of Crude Oil-Brine-Rock Interactions and Wettability Alteration during Low-Salinity Waterflooding. Fuel 250, 117–131. doi:10.1016/j.fuel.2019.02.019
King, F. (2007). Overview of a Carbon Steel Container Corrosion Model for a Deep Geological Repository in Sedimentary Rock. Nuclear Waste Management Organization Report No.: NWMO TR-2007-01.
Kluge, J., and Ziefle, M. (2016). “As Simple as Possible and as Complex as Necessary,” in International Conference on HCI in Business, Government, and Organizations (Cham: Springer), 171–182. doi:10.1007/978-3-319-39399-5_17
Koehn, D., Piazolo, S., Sachau, T., and Toussaint, R. (2020). Fracturing and Porosity Channeling in Fluid Overpressure Zones in the Shallow Earth’s Crust. Geofluids 2020, 7621759. doi:10.1155/2020/7621759
Kong, Y., Pang, Z., Shao, H., Hu, S., and Kolditz, O. (2014). Recent Studies on Hydrothermal Systems in China: a Review. Geotherm. Energy 2 (1), 19. doi:10.1186/s40517-014-0019-8
Korbøl, R., and Kaddour, A. (1995). Sleipner Vest CO2 Disposal - Injection of Removed CO2 into the Utsira Formation. Energy Convers. Manag. 36, 509–512.
Kowalewski, S., Borg, A., Kluge, J., Himmel, S., Trevisan, B., Eraßme, D., et al. (2014). Modelling the Influence of Human Factors on the Perception of Renewable Energies. Taking Geothermics as an ExampleAdvances in Human Factors, Software and System Engineering, 155–162.
Kumari, W. G. P., Ranjith, P. G., Perera, M. S. A., Shao, S., Chen, B. K., Lashin, A., et al. (2017). Mechanical Behaviour of Australian Strathbogie Granite under In-Situ Stress and Temperature Conditions: An Application to Geothermal Energy Extraction. Geothermics 65, 44–59. doi:10.1016/j.geothermics.2016.07.002
Kwiatek, G., Saarno, T., Ader, T., Bluemle, F., Bohnhoff, M., Chendorain, M., et al. (2019). Controlling Fluid-Induced Seismicity during a 6.1-Km-Deep Geothermal Stimulation in Finland. Sci. Adv. 5 (5), eaav7224. doi:10.1126/sciadv.aav7224
Lagaly, G. (2006). Handb. Clay Sci. Dev. Clay Sci. “Chapter 5 Colloid Clay Science,”. Editors F. Bergaya, B. K. G. Theng, and G. Lagaly, 1, 141–245. doi:10.1016/S1572-4352(05)01005-6
Lai, J., Wang, G., Wang, Z., Chen, J., Pang, X., Wang, S., et al. (2018). A Review on Pore Structure Characterization in Tight Sandstones. Earth-Science Rev. 177, 436–457. doi:10.1016/j.earscirev.2017.12.003
Lake, R. D., Northmore, K. J., Dean, M. T., and Tragheim, D. G. (1992). Leeds: A Geological Background for Planning and Development. Keyworth, Nottingham: British Geological Survey.
Lander, R. H., and Walderhaug, O. (1999). Predicting Porosity through Simulating Sandstone Compaction and Quartz Cementation. AAPG Bull. 83 (3), 433–449. doi:10.1306/00aa9bc4-1730-11d7-8645000102c1865d
Lankof, L., Polański, K., Ślizowski, J., and Tomaszewska, B. (2016). Possibility of Energy Storage in Salt Caverns. drill 33 (2), 405–415. doi:10.7494/drill.2016.33.2.405
Lanyon, G. W., Marschall, P., Trick, T., de La Vaissière, R., Shao, H., and Leung, H. (2009). “January. Hydromechanical Evolution and Self-Sealing of Damage Zones Around a Microtunnel in a Claystone Formation of the Swiss Jura Mountains,” in 43rd US Rock Mechanics Symposium & 4th US-Canada Rock Mechanics Symposium, Asheville, North Carolina, June 28–July 1, 2009 (American Rock Mechanics Association).
Lash, G. G., and Engelder, T. (2005). An Analysis of Horizontal Microcracking during Catagenesis: Example from the Catskill Delta Complex. Bulletin 89 (11), 1433–1449. doi:10.1306/05250504141
Laubach, S. E., Lander, R. H., Criscenti, L. J., Anovitz, L. M., Urai, J. L., Pollyea, R. M., et al. (2019). The Role of Chemistry in Fracture Pattern Development and Opportunities to Advance Interpretations of Geological Materials. Rev. Geophys. 57 (3), 1065–1111. doi:10.1029/2019rg000671
Laubach, S. E., Marrett, R. A., Olson, J. E., and Scott, A. R. (1998). Characteristics and Origins of Coal Cleat: a Review. Int. J. Coal Geol. 35 (1-4), 175–207. doi:10.1016/s0166-5162(97)00012-8
Law, R., Bridgland, D., Nicholson, D., and Chendorain, M. (2015). “April. Heat Extraction from Deep Single Wells,” in Proceedings World Geothermal Congress, 19–25.
Lecocq, T., Hicks, S. P., Van Noten, K., Van Wijk, K., Koelemeijer, P., De Plaen, R. S. M., et al. (2020). Global Quieting of High-Frequency Seismic Noise Due to COVID-19 Pandemic Lockdown Measures. Science 369 (6509), 1338–1343. doi:10.1126/science.abd2438
Lee, H. P., Olson, J. E., Holder, J., Gale, J. F. W., and Myers, R. D. (2015). The Interaction of Propagating Opening Mode Fractures with Preexisting Discontinuities in Shale. J. Geophys. Res. Solid Earth 120 (1), 169–181. doi:10.1002/2014jb011358
Lewis, S., and Holness, M. (1996). Equilibrium Halite-H2o Dihedral Angles: High Rock-Salt Permeability in the Shallow Crust? Geol 24, 431–434. doi:10.1130/0091-7613(1996)024<0431:ehhoda>2.3.co;2
Li, J. F., Li, X. M., Lin, J. W., Liu, J., Wang, L. C., and Gao, B. Z. (2012). An Analysis of Compositions of the Deposits of Geothermal Fluid in Tianjin. Hydrogeology Eng. Geol. 39, 137–144.
Li, M., Li, G., Yang, L., Dang, X., Zhao, C., Hou, G., et al. (2007). Numerical Modeling of Geothermal Groundwater Flow in Karst Aquifer System in Eastern Weibei, Shaanxi Province, China. Sci. China Ser. D Earth Sci. 50 (1), 36–41. doi:10.1007/s11430-007-5020-7
Li, Z., Dong, M., Li, S., and Huang, S. (2006). CO2 Sequestration in Depleted Oil and Gas Reservoirs-Caprock Characterization and Storage Capacity. Energy Convers. Manag. 47 (11–12), 1372–1382. doi:10.1016/j.enconman.2005.08.023
Lima, B. E. M., and De Ros, L. F. (2019). Deposition, Diagenetic and Hydrothermal Processes in the Aptian Pre-salt Lacustrine Carbonate Reservoirs of the Northern Campos Basin, Offshore Brazil. Sediment. Geol. 383, 55–81. doi:10.1016/j.sedgeo.2019.01.006
Liu, Z., and Zhao, J. (2000). Contribution of Carbonate Rock Weathering to the Atmospheric CO 2 Sink. Environ. Geol. 39 (9), 1053–1058. doi:10.1007/s002549900072
Lo Ré, C., Kaszuba, J. P., Moore, J. N., and McPherson, B. J. (2014). Fluid-rock Interactions in CO2-saturated, Granite-Hosted Geothermal Systems: Implications for Natural and Engineered Systems from Geochemical Experiments and Models. Geochimica Cosmochimica Acta 141, 160–178. doi:10.1016/j.gca.2014.06.015
Lokhorst, A., and Wildenborg, T. (2005). Introduction on CO2 Geological Storage - Classification of Storage Options. Oil Gas Sci. Technol. - Rev. IFP 60, 513–515. doi:10.2516/ogst:2005033
Lønøy, A. (2006). Making Sense of Carbonate Pore Systems. Bulletin 90, 1381–1405. doi:10.1306/03130605104
Lopez, S., Hamm, V., Le Brun, M., Schaper, L., Boissier, F., Cotiche, C., et al. (2010). 40 Years of Dogger Aquifer Management in Ile-de-France, Paris Basin, France. Geothermics 39, 339–356. doi:10.1016/j.geothermics.2010.09.005
Loredo, C., Ordóñez, A., Garcia-Ordiales, E., Álvarez, R., Roqueñi, N., Cienfuegos, P., et al. (2017). Hydrochemical Characterization of a Mine Water Geothermal Energy Resource in NW Spain. Sci. Total Environ. 576, 59–69. doi:10.1016/j.scitotenv.2016.10.084
Lu, J., Kharaka, Y. K., Thordsen, J. J., Horita, J., Karamalidis, A., Griffith, C., et al. (2012). CO2-rock-brine Interactions in Lower Tuscaloosa Formation at Cranfield CO2 Sequestration Site, Mississippi, U.S.A. Chem. Geol. 291, 269–277. doi:10.1016/j.chemgeo.2011.10.020
Lund, J., Sanner, B., Rybach, L., Curtis, R., and Hellström, G. (2004). Geothermal (Ground-source) Heat Pumps: a World Overview. Geo-Heat Cent. Q. Bull. 25.
Luo, J. (2014). Experimental Measurements and Numerical Modeling of a Ground Source Heat Pump SystemDoctoral dissertation. Erlangen, Germany: Friedrich-Alexander-Universität Erlangen-Nürnberg FAU.
Macgregor, D. S. (1996). Factors Controlling the Destruction or Preservation of Giant Light Oilfields. Pet. Geosci. 2, 197–217. doi:10.1144/petgeo.2.3.197
Majer, E. L., Baria, R., Stark, M., Oates, S., Bommer, J., Smith, B., et al. (2007). Induced Seismicity Associated with Enhanced Geothermal Systems. Geothermics 36 (3), 185–222. doi:10.1016/j.geothermics.2007.03.003
Major, J. R., Eichhubl, P., Dewers, T. A., and Olson, J. E. (2018). Effect of CO2-brine-rock Interaction on Fracture Mechanical Properties of CO2 Reservoirs and Seals. Earth Planet. Sci. Lett. 499, 37–47. doi:10.1016/j.epsl.2018.07.013
Marschall, P., Trick, T., Lanyon, G. W., Delay, J., and Shao, H. (2008). “January. Hydro-Mechanical Evolution of Damaged Zones Around a Microtunnel in a Claystone Formation of the Swiss Jura Mountains,” in The 42nd US Rock Mechanics Symposium (USRMS) (American Rock Mechanics Association).
Martinez, J., Johnson, K., and Neal, J. (1998). Sinkholes in Evaporite Rocks. Amer. Sci. 86 (1), 38–51. doi:10.1511/1998.1.38
Matray, J. M., Savoye, S., and Cabrera, J. (2007). Desaturation and Structure Relationships Around Drifts Excavated in the Well-Compacted Tournemire's Argillite (Aveyron, France). Eng. Geol. 90 (1-2), 1–16. doi:10.1016/j.enggeo.2006.09.021
Matter, J. M., Broecker, W. S., Stute, M., Gislason, S. R., Oelkers, E. H., Stefánsson, A., et al. (2009). Permanent Carbon Dioxide Storage into Basalt: The CarbFix Pilot Project, Iceland. Energy Procedia 1 (1), 3641–3646. doi:10.1016/j.egypro.2009.02.160
McCartney, R. (1987). Hot Dry Rock Geothermal Systems: Geochemical Applications of Transient Field Experiments. Geothermics 16 (4), 419–428. doi:10.1016/0375-6505(87)90022-8
Ménager, M.-T., Petit, J.-C., and Brocandel, M. (1992). The Migration of Radionuclides in Granite: a Review Based on Natural Analogues. Appl. Geochem. 7, 217–238. doi:10.1016/S0883-2927(09)80078-4
Menefee, A. H., Welch, N. J., Frash, L. P., Hicks, W., Carey, J. W., and Ellis, B. R. (2020). Rapid Mineral Precipitation during Shear Fracturing of Carbonate-Rich Shales. J. Geophys. Res. Solid Earth 125 (6), e2019JB018864. doi:10.1029/2019jb018864
Menéndez, J., Ordóñez, A., Álvarez, R., and Loredo, J. (2019). Energy from Closed Mines: Underground Energy Storage and Geothermal Applications. Renew. Sustain. Energy Rev. 108, 498–512. doi:10.1016/j.rser.2019.04.007
Menezes, C. P., Bezerra, F. H. R., Balsamo, F., Mozafari, M., Vieira, M. M., Srivastava, N. K., et al. (2019). Hydrothermal Silicification along Faults Affecting Carbonate-Sandstone Units and its Impact on Reservoir Quality, Potiguar Basin, Brazil. Mar. Petroleum Geol. 110, 198–217. doi:10.1016/j.marpetgeo.2019.07.018
Metcalfe, R., Thatcher, K., Towler, G., Paulley, A., and Eng, J. (2017). Sub-surface Risk Assessment for the Endurance CO2 Store of the White Rose Project, UK. Energy Procedia 114, 4313–4320. doi:10.1016/j.egypro.2017.03.1578
Metcalfe, R., Watson, S. P., Rees, J. H., Humphreys, P., and King, F. (2008). Gas Generation and Migration from a Deep Geological Repository for Radioactive Waste A Review of Nirex/NDA’s Work.
Michie, E. A. H., Cooke, A. P., Kaminskaite, I., Stead, J. C., Plenderleith, G. E., Tobiss, S. D., et al. (2020a). Key Controls on the Hydraulic Properties of Fault Rocks in Carbonates. Pet. Geosci. doi:10.1144/petgeo2020-034
Michie, E. A. H., Kaminskaite, I., Cooke, A. P., Fisher, Q. J., Yielding, G., and Tobiss, S. D. (2020b). Along-Strike Permeability Variation in Carbonate-Hosted Fault Zones. J. Struct. Geol., 104236.
Minissale, A. (2004). Origin, Transport and Discharge of CO2 in Central Italy. Earth-Science Rev. 66 (1-2), 89–141. doi:10.1016/j.earscirev.2003.09.001
Missana, T., Alonso, U., Albarran, N., García-Gutiérrez, M., and Cormenzana, J. L. (2011). Analysis of Colloids Erosion from the Bentonite Barrier of a High Level Radioactive Waste Repository and Implications in Safety Assessment. Phys. Chem. Earth, Parts A/B/C 36 (17-18), 1607–1615. doi:10.1016/j.pce.2011.07.088
Missana, T., García-Gutiérrez, M., Alonso, U., and Mingarro, M. (2006). On Radionuclide Retention Mechanisms in Fractured Geologic Media. J. Iber. Geol. 32 (1), 55–77.
M. J. Apted, and J. Ahn (Editors) (2017). Geological Repository Systems for Safe Disposal of Spent Nuclear Fuels and Radioactive Waste (Sawston, UK: Woodhead Publishing).
Moeck, I. S. (2014). Catalog of Geothermal Play Types Based on Geologic Controls. Renew. Sustain. Energy Rev. 37, 867–882. doi:10.1016/j.rser.2014.05.032
Montanari, D., Minissale, A., Doveri, M., Gola, G., Trumpy, E., Santilano, A., et al. (2017). Geothermal Resources within Carbonate Reservoirs in Western Sicily (Italy): A Review. Earth-Science Rev. 169, 180–201. doi:10.1016/j.earscirev.2017.04.016
Montes, H. G., Duplay, J., Martinez, L., Escoffier, S., and Rousset, D. (2004). Structural Modifications of Callovo-Oxfordian Argillite under Hydration/dehydration Conditions. Appl. Clay Sci. 25, 187–194. doi:10.1016/j.clay.2003.10.004
Montori, J., Saaltink, M. V., and Soler, J. M. (2008). Reactive Transport Modeling of the Effect of Hyperalkaline Solutions along a Fracture at the ONKALO Site (No. POSIVA-WR--08-14). Posiva Oy.
Moya, D., Aldás, C., and Kaparaju, P. (2018). Geothermal Energy: Power Plant Technology and Direct Heat Applications. Renew. Sustain. Energy Rev. 94, 889–901. doi:10.1016/j.rser.2018.06.047
Murray, R. C. (1964). Origin and Diagenesis of Gypsum and Anhydrite. J. Sed. Res. 34, 512–523. doi:10.1306/74d710d2-2b21-11d7-8648000102c1865d
Natarajan, N., and Kumar, G. S. (2010). Radionuclide and Colloid Co-transport in a Coupled Fracture-Skin-Matrix System. Colloids Surfaces A Physicochem. Eng. Aspects 370 (1-3), 49–57. doi:10.1016/j.colsurfa.2010.08.045
Natarajan, N., and Suresh Kumar, G. (2012). Evolution of Fracture Permeability Due to Co-colloidal Bacterial Transport in a Coupled Fracture-Skin-Matrix System. Geosci. Front. 3 (4), 503–514. doi:10.1016/j.gsf.2011.12.003
NDA (2016a). Geological Disposal: Waste Package Evolution Status Report. NDA Report no. DSSC/451/01.
NDA (2016b). Geological Disposal: Generic Post-closure Performance Modelling. NDA Report no. NDA/RWM/138.
Ng, C., Paraskevopoulou, C., and Shaw, N. (2019). Stability Analysis of Shafts Used for Minewater Heat Recovery. Geotech. Geol. Eng. 37 (6), 5245–5268. doi:10.1007/s10706-019-00978-y
Niederau, J., Gomez, S., Ebigbo, A., Inversi, B., Marquart, G., and Scrocca, D. (2015). “Hydrothermal Simulation of a Fractured Carbonate Reservoir in Southern Italy and Automated Detections of Optimal Positions for Geothermal Doublet Installations,” in 2015 EGU General Assembly 2015, Vienna, Austria (EGUGA), April 12–17, 2015, 12090.
Nielsen, L. H., Mathiesen, A., and Bidstrup, T. (2004). Geothermal Energy in Denmark. GEUS Bull. 4, 17–20. doi:10.34194/geusb.v4.4771
Norden, B., and Foster, A. (2006). Thermal Conductivity and Radiogenic Heat Production of Sedimentary and Magmatic Rocks in the Northeast German Basin. Bulletin 90 (6), 939–962. doi:10.1306/01250605100
Okamoto, J., Fujita, T., Hara, K., and Sasaki, N. (1991). “Effect of Heat from High-Level Waste on Performance of Deep Geological Repository,” in Proceedings of the third international conference on nuclear fuel reprocessing and waste management, RECOD'91, Sendai, Japan, April 14-18, 1991.
Okech, R. R., Liu, X., Falcone, G., and Teodoriu, C. (2015). “Unconventional Completion Design for Deep Geothermal Wells,” in SPE Latin American and Caribbean Petroleum Engineering Conference, Quito, Ecuador, 18 - 20 Nov 2015. doi:10.2118/177228-ms
Olivella, S., and Alonso, E. E. (2004). Modelling Gas Flow through Deformable Fractured Rocks. Elsevier Geo-Engineering Book Ser. 2, 31–36. doi:10.1016/s1571-9960(04)80020-x
Olivella, S., Castagna, S., Alonso, E. E., and Lloret, A. (2011). Porosity Variations in Saline Media Induced by Temperature Gradients: Experimental Evidences and Modelling. Transp. Porous Med. 90 (3), 763–777. doi:10.1007/s11242-011-9814-x
Ougier-Simonin, A., Renard, F., Boehm, C., and Vidal-Gilbert, S. (2016). Microfracturing and Microporosity in Shales. Earth-Science Rev. 162, 198–226. doi:10.1016/j.earscirev.2016.09.006
Packard, J. J., Al-Aasm, I., Samson, I., Berger, Z., and Davies, J. (2001). A Devonian Hydrothermal Chert Reservoir: the 225 Bcf Parkland Field, British Columbia, Canada. AAPG Bull. 85 (1), 51–84. doi:10.1306/8626c75d-173b-11d7-8645000102c1865d
Padin, A., Tutuncu, A. N., and Sonnenberg, S. (2014). On the Mechanisms of Shale Microfracture Propagation. SPE Hydraulic Fracturing Technology Conference. Soc. Pet. Eng.
Palmer, A. N. (1991). Origin and Morphology of Limestone Caves. Geol. Soc. Am. Bull. 103, 1–21. doi:10.1130/0016-7606(1991)103<0001:oamolc>2.3.co;2
Pandey, S. N., Chaudhuri, A., and Kelkar, S. (2017). A Coupled Thermo-Hydro-Mechanical Modeling of Fracture Aperture Alteration and Reservoir Deformation during Heat Extraction from a Geothermal Reservoir. Geothermics 65, 17–31. doi:10.1016/j.geothermics.2016.08.006
Parisio, F., Vilarrasa, V., Wang, W., Kolditz, O., and Nagel, T. (2019). The Risks of Long-Term Re-injection in Supercritical Geothermal Systems. Nat. Commun. 10 (1), 1–11. doi:10.1038/s41467-019-12146-0
Pasikki, R. G., and Pasaribu, H. (2014). “Application of Hydraulic Stimulation to Improve Well Injectivity,” in Presented at the Indonesia International Geothermal Convention & Exhibition 2014 (Indonesia: Jakarta Convention Center), 4–6.
Piazolo, S., Bons, P. D., Griera, A., Llorens, M.-G., Gomez-Rivas, E., Koehn, D., et al. (2019). A Review of Numerical Modelling of the Dynamics of Microstructural Development in Rocks and Ice: Past, Present and Future. J. Struct. Geol. 125, 111–123. doi:10.1016/j.jsg.2018.05.025
Pineda, J. A., Alonso, E. E., and Romero, E. (2014). Environmental Degradation of Claystones. Géotechnique 64 (1), 64–82. doi:10.1680/geot.13.p.056
Plumlee, G. S. (1999). The Environmental Geochemistry of Mineral Deposits. Colorado, US: Society of Economic Geologists, 71–116.
Popp, T., Salzer, K., and Minkley, W. (2008). Influence of Bedding Planes to EDZ-Evolution and the Coupled HM Properties of Opalinus Clay. Phys. Chem. Earth, Parts A/B/C 33, S374–S387. doi:10.1016/j.pce.2008.10.018
Poros, Z., Jagniecki, E., Luczaj, J., Kenter, J., Gal, B., Correa, T. S., et al. (2017). Origin of Silica in Pre-salt Carbonates. Angola: Kwanza Basin.
Preene, M., and Younger, P. L. (2014). Can You Take the Heat? - Geothermal Energy in Mining. Min. Technol. 123 (2), 107–118. doi:10.1179/1743286314y.0000000058
Pruess, K. (2006). Enhanced Geothermal Systems (EGS) Using CO2 as Working Fluid-A Novel Approach for Generating Renewable Energy with Simultaneous Sequestration of Carbon. Geothermics 35, 351–367. doi:10.1016/j.geothermics.2006.08.002
Pruess, K. (2005). Numerical Studies of Fluid Leakage from a Geologic Disposal Reservoir for CO2 Show Self-Limiting Feedback between Fluid Flow and Heat Transfer. Geophys. Res. Lett. 32 (14). doi:10.1029/2005gl023250
Putnis, A., Oelkers, E. H., and Schott, J. (2009). 3. Mineral Replacement Reactions. Thermodyn. Kinet. water-rock Interact. 70, 87–124. doi:10.1515/9781501508462-005
Pyrak-Nolte, L. J., DePaolo, D. J., and Pietraß, T. (2015). Controlling Subsurface Fractures and Fluid Flow: A Basic Research Agenda. Washington, DC: Office of Energy Efficiency & Renewable Energy.
Qian, J., Wang, L., Ma, L., Lu, Y., Zhao, W., and Zhang, Y. (2016). Multivariate Statistical Analysis of Water Chemistry in Evaluating Groundwater Geochemical Evolution and Aquifer Connectivity Near a Large Coal Mine, Anhui, China. Environ. Earth Sci. 75 (9), 747. doi:10.1007/s12665-016-5541-5
Rad, F. M., Fung, A. S., and Rosen, M. A. (2017). An Integrated Model for Designing a Solar Community Heating System with Borehole Thermal Storage. Energy Sustain. Dev. 36, 6–15. doi:10.1016/j.esd.2016.10.003
Ragheb, M. (2011). Management of Radioactive Waste. Nuclear Plasma and Radiation Science (Part III).
Ranathunga, A. S., Ranjith, P. G., and Perera, M. S. A. (2017). “Challenges and Issues for CO2 Storage in Deep Coal Seams,” in Rock Mechanics and Engineering Volume 4: Excavation, Support and Monitoring (Florida, US: CRC Press), 87–119.
Rattle, I., Van Alstine, J., Peterson, E., Shaw, N., and Piazolo, S. (2020). Using the Underground to Fight Climate Change. Policy Leeds Brief. 2. SRI 29.
Reinecker, J., Hochschild, T., Kraml, M., Löschan, G., and Kreuter, H. (2019). “Experiences and Challenges in Geothermal Exploration in the Upper Rhine Graben,” in Proceedings of European Geothermal Congress in Den Haag, Hauge, Netherlands, 11-14 June 2019.
Richards, H. G., Savage, D., and Andrews, J. N. (1992). Granite-water Reactions in an Experimental Hot Dry Rock Geothermal Reservoir, Rosemanowes Test Site, Cornwall, U.K. Appl. Geochem. 7 (3), 193–222. doi:10.1016/0883-2927(92)90038-5
Rizzo, P., Cappadonia, C., Rotigliano, E., Iacumin, P., Sanangelantoni, A. M., Zerbini, G., et al. (2020). Hydrogeological Behaviour and Geochemical Features of Waters in Evaporite-Bearing Low-Permeability Successions: A Case Study in Southern Sicily, Italy. Appl. Sci. 10 (22), 8177. doi:10.3390/app10228177
Roberts, J. J., and Stalker, L. (2017). What Have We Learned about CO2 Leakage from Field Injection Tests? Energy Procedia 114, 5711–5731. doi:10.1016/j.egypro.2017.03.1710
Rosborg, B., and Werme, L. (2008). The Swedish Nuclear Waste Program and the Long-Term Corrosion Behaviour of Copper. J. Nucl. Mater. 379 (1-3), 142–153. doi:10.1016/j.jnucmat.2008.06.025
Rostom, F., Røyne, A., Dysthe, D. K., and Renard, F. (2013). Effect of Fluid Salinity on Subcritical Crack Propagation in Calcite. Tectonophysics 583, 68–75. doi:10.1016/j.tecto.2012.10.023
Salimzadeh, S., Paluszny, A., and Zimmerman, R. W. (2018). Effect of Cold CO2 Injection on Fracture Apertures and Growth. Int. J. Greenh. Gas Control 74, 130–141. doi:10.1016/j.ijggc.2018.04.013
Sanford, W. E., and Konikow, L. F. (1989). Simulation of Calcite Dissolution and Porosity Changes in Saltwater Mixing Zones in Coastal Aquifers. Water Resour. Res. 25 (4), 655–667. doi:10.1029/wr025i004p00655
Savage, D., Noy, D., and Mihara, M. (2002). Modelling the Interaction of Bentonite with Hyperalkaline Fluids. Appl. Geochem. 17, 207–223. doi:10.1016/S0883-2927(01)00078-6
Savage, D., and Rochelle, C. A. (1993). Modelling Reactions between Cement Pore Fluids and Rock: Implications for Porosity Change. J. Contam. Hydrology 13 (1-4), 365–378. doi:10.1016/0169-7722(93)90071-y
Schmoker, J. W., and Halley, R. B. (1982). Carbonate Porosity versus Depth: a Predictable Relation for South Florida. AAPG Bull. 66 (12), 2561–2570. doi:10.1306/03b5ac73-16d1-11d7-8645000102c1865d
Schubnel, A., Fortin, J., Burlini, L., and Guéguen, Y. (2005). Damage and Recovery of Calcite Rocks Deformed in the Cataclastic Regime. Geol. Soc. Lond. Spec. Publ. 245, 203–221. doi:10.1144/gsl.sp.2005.245.01.10
Schumacher, S., and Schulz, R. (2013). Effectiveness of Acidizing Geothermal Wells in the South German Molasse Basin. Geoth. Energ. Sci. 1 (1), 1–11. doi:10.5194/gtes-1-1-2013
Schwartz, M. O. (2012). Modelling Groundwater Contamination above a Nuclear Waste Repository at Gorleben, Germany. Hydrogeol. J. 20 (3), 533–546. doi:10.1007/s10040-011-0825-z
Scourfield, S. J., Kent, J. E., Wickham, S. M., Nieminen, M., Clarke, S., and Frasca, B. (2020). “Thermal Treatment for Radioactive Waste Minimisation and Hazard Reduction: Overview and Summary of the EC THERAMIN Project,” in IOP Conference Series: Materials Science and Engineering, April, 2020 (IOP Publishing), Vol. 818 (1), 012001.
Segall, P., and Pollard, D. D. (1983). Nucleation and Growth of Strike Slip Faults in Granite. J. Geophys. Res. 88 (B1), 555–568. doi:10.1029/jb088ib01p00555
Sellin, P., and Leupin, O. X. (2013). The Use of Clay as an Engineered Barrier in Radioactive-Waste Management - A Review. Clays Clay Min. 61 (6), 477–498. doi:10.1346/ccmn.2013.0610601
Setiawan, F. A., Rahayuningsih, E., Petrus, H. T. B. M., Nurpratama, M. I., and Perdana, I. (2019). Kinetics of Silica Precipitation in Geothermal Brine with Seeds Addition: Minimizing Silica Scaling in a Cold Re-injection System. Geotherm. Energy 7 (1), 1–16. doi:10.1186/s40517-019-0138-3
Shahbazi, K., Zarei, A. H., Shahbazi, A., and Tanha, A. A. Z. (2020). Investigation of Production Depletion Rate Effect on the Near-Wellbore Stresses in the Two Iranian Southwest Oilfields. Petroleum Res. doi:10.1016/j.ptlrs.2020.07.002
Shao, S., Ranjith, P. G., Wasantha, P. L. P., and Chen, B. K. (2015). Experimental and Numerical Studies on the Mechanical Behaviour of Australian Strathbogie Granite at High Temperatures: an Application to Geothermal Energy. Geothermics 54, 96–108. doi:10.1016/j.geothermics.2014.11.005
Sharma, S., Van Gent, D., Burke, M., and Stelfox, L. (2017). The Australian South West Hub Project: Developing a Storage Project in Unconventional Geology. Energy Procedia 114, 4524–4536. doi:10.1016/j.egypro.2017.03.1569
Shaw, D. B., and Weaver, C. E. (1965). The Mineralogical Composition of Shales. J. Sediment. Pet-rol. 35, 213–222. doi:10.1306/74d71221-2b21-11d7-8648000102c1865d
Shi, J. Q., and Durucan, S. (2005). CO2Storage in Caverns and Mines. Oil Gas Sci. Technol. - Rev. IFP 60 (3), 569–571. doi:10.2516/ogst:2005039
Sigfússon, B., and Uihlein, A. (2015). JRC Geothermal Energy Status Report: Technology, Market and Economic Aspects of Geothermal Energy in Europe. Luxembourg: European Union.
Simmons, C. J., and Freiman, S. W. (1981). Effect of Corrosion Processes on Subcritical Crack Growth in Glass. J Am. Ceram. Soc. 64, 683–686. doi:10.1111/j.1151-2916.1981.tb15870.x
Siren, T. (2015). Excavation Damage Zones, Fracture Mechanics Simulation and in Situ Strength of Migmatitic Gneiss and Pegmatitic Granite at the Nuclear Waste Disposal Site in Olkiluoto. Western Finland: Department of Civil and Environmental Engineering.
Sliaupa, S., Motuza, G., Motuza, V., Puronas, V., Korabliova, L., and Ciuraite, K. (2010). “Geothermal Potential of Hot Granites of Lithuania,” in Proceedings World Geothermal Congress, Bali, Indonesia, 25-29 April 2010.
Smith, M. M., Sholokhova, Y., Hao, Y., and Carroll, S. A. (2013). Evaporite Caprock Integrity: An Experimental Study of Reactive Mineralogy and Pore-Scale Heterogeneity during Brine-CO2 Exposure. Environ. Sci. Technol. 47 (1), 262–268. doi:10.1021/es3012723
Smith, S. L. (2015). The Impact of Hyper-Alkaline Fluids from a Geological Radioactive Waste Repository on the Biological and Physical Characteristics of the Host Rock Environment. Ph D Thesis (Manchester, England: The University of Manchester).
Soltanzadeh, H., and Hawkes, C. D. (2008). Semi-analytical Models for Stress Change and Fault Reactivation Induced by Reservoir Production and Injection. J. Petroleum Sci. Eng. 60 (2), 71–85. doi:10.1016/j.petrol.2007.05.006
Sørensen, P. A., and Schmidt, T. (2018). “Design and Construction of Large Scale Heat Storages for District Heating in Denmark,” in 14th International Conference on Energy Storage, Adana, Turkey, 25-28 April 2018.
Spearing, A. J. S. (1994). An Overview of Coal Mining in Poland. J. South Afr. Inst. Min. Metallurgy 94 (8), 215–217.
Spycher, N. F., Sonnenthal, E. L., and Apps, J. A. (2003). Fluid Flow and Reactive Transport Around Potential Nuclear Waste Emplacement Tunnels at Yucca Mountain, Nevada. J. Contam. Hydrology 62-63, 653–673. doi:10.1016/s0169-7722(02)00183-3
Stáhl, G., Pátzay, G., Weiser, L., and Kálmán, E. (2000). Study of Calcite Scaling and Corrosion Processes in Geothermal Systems. Geothermics 29 (1), 105–119. doi:10.1016/s0375-6505(99)00052-8
Stephenson, M. H., Ringrose, P., Geiger, S., Bridden, M., and Schofield, D. (2019). Geoscience and Decarbonization: Current Status and Future Directions. Pet. Geosci. 25 (4), 501–508. doi:10.1144/petgeo2019-084
Su, Y., Yang, F., Wang, B., Jia, Z., and Duan, Z. (2018). Reinjection of Cooled Water into Sandstone Geothermal Reservoirs in China: a Review. Geosci. J. 22 (1), 199–207. doi:10.1007/s12303-017-0019-3
Sun, Q., Lü, C., Cao, L., Li, W., Geng, J., and Zhang, W. (2016). Thermal Properties of Sandstone after Treatment at High Temperature. Int. J. Rock Mech. Min. Sci. 85, 60–66. doi:10.1016/j.ijrmms.2016.03.006
Suresh Kumar, G. (2015). Subsurface Transport of Nuclear Wastes in the Indian Subcontinent. ISH J. Hydraulic Eng. 21 (2), 162–176. doi:10.1080/09715010.2014.984249
Swarbrick, R. E., Jenkins, S. J., Scott, D. S., and Riddle, G. (2013). The Role of Pressure in Carbon Capture and Storage (CCS). Geol. Storage Carbon Dioxide (CO2) 2013, 97–110e. doi:10.1533/9780857097279.1.97
Swarbrick, R. E., Lahann, R. W., O'Connor, S. A., and Mallon, A. J. (2010). Role of the Chalk in Development of Deep Overpressure in the Central North Sea. Pet. Geol. Conf. Ser. 7, 493–507. doi:10.1144/0070493
Swarbrick, R. E., and Osborne, M. J. (1998). Mechanisms that Generate Abnormal Pressures: an Overview. Abnorm. Press. Hydrocarb. Environ. AAPG Mem. 70, 13–34.
Swarbrick, R. E., Seldon, B., and Mallon, A. J. (2005). Modelling the Central North Sea Pressure History. Pet. Geol. Conf. Ser. 6, 1237–1245. doi:10.1144/0061237
Takahashi, T., and Hashida, T. (2004). Microcrack Formation and Fracture Characteristics in Granite under Supercritical Water Conditions. Elsevier Geo-Engineering Book Ser. 2, 685–690. doi:10.1016/s1571-9960(04)80119-8
Taron, J., and Elsworth, D. (2009). Thermal-hydrologic-mechanical-chemical Processes in the Evolution of Engineered Geothermal Reservoirs. Int. J. Rock Mech. Min. Sci. 46, 855–864. doi:10.1016/j.ijrmms.2009.01.007
Taylor, O. D. S., Lester, A. P., Lee, T. A., and McKenna, M. H. (2018). Can Repetitive Small Magnitude-Induced Seismic Events Actually Cause Damage? Adv. Civ. Eng.
Techer, I., Khoury, H. N., Salameh, E., Rassineux, F., Claude, C., Clauer, N., et al. (2006). Propagation of High-Alkaline Fluids in an Argillaceous Formation: Case Study of the Khushaym Matruk Natural Analogue (Central Jordan). J. Geochem. Explor. 90 (1-2), 53–67. doi:10.1016/j.gexplo.2005.09.004
Todd, F., McDermott, C., Harris, A. F., Bond, A., and Gilfillan, S. (2019). Coupled Hydraulic and Mechanical Model of Surface Uplift Due to Mine Water Rebound: Implications for Mine Water Heating and Cooling Schemes. Scott. J. Geol. 55, 124–133. doi:10.1144/sjg2018-028
Trautz, R. C., Pugh, J. D., Varadharajan, C., Zheng, L., Bianchi, M., Nico, P. S., et al. (2013). Effect of Dissolved CO2 on a Shallow Groundwater System: a Controlled Release Field Experiment. Environ. Sci. Technol. 47, 298–305. doi:10.1021/es301280t
Tsang, C.-F., Bernier, F., and Davies, C. (2005). Geohydromechanical Processes in the Excavation Damaged Zone in Crystalline Rock, Rock Salt, and Indurated and Plastic Clays-In the Context of Radioactive Waste Disposal. Int. J. Rock Mech. Min. Sci. 42, 109–125. doi:10.1016/j.ijrmms.2004.08.003
Tsang, C. F., Barnichon, J. D., Birkholzer, J., Li, X. L., Liu, H. H., and Sillen, X. (2012). Coupled Thermo-Hydro-Mechanical Processes in the Near Field of a High-Level Radioactive Waste Repository in Clay Formations. Int. J. Rock Mech. Min. Sci. 49, 31–44. doi:10.1016/j.ijrmms.2011.09.015
Ukar, E., Baqués, V., Laubach, S. E., and Marrett, R. (2020). The Nature and Origins of Decametre-Scale Porosity in Ordovician Carbonate Rocks, Halahatang Oilfield, Tarim Basin, China. J. Geol. Soc. 177 (5), 1074–1091. doi:10.1144/jgs2019-156
Urai, J. L., Spiers, C. J., Zwart, H. J., and Lister, G. S. (1986). Weakening of Rock Salt by Water during Long-Term Creep. Nature 324, 554–557. doi:10.1038/324554a0
Urquhart, A. S. M. (2011). Structural Controls on CO2 Leakage and Diagenesis in a Natural Long-Term Carbon Sequestration Analogue: Little Grand Wash Fault, Utah. Austin: Department of Geological Sciences, Jackson School of Geosciences, University of Texas at Austin, 437.
Vaitiekūnas, R. (2012). Klaipedos Demonstration Geothermal Plant. Available at: https://docplayer.lt/156684670-2012-m-kovo-23-d-uab-geoterma.html (Accessed December 14th, 2020).
Van Aardt, J. H. P., and Visser, S. (1977). Calcium Hydroxide Attack on Feldspars and Clays: Possible Relevance to Cement-Aggregate Reactions. Cem. Concr. Res. 7, 643–648. doi:10.1016/0008-8846(77)90046-1
Van Driessche, A. E. S., Stawski, T. M., Benning, L. G., and Kellermeier, M. (2017). “Calcium Sulfate Precipitation throughout its Phase Diagram,” in New Perspectives on Mineral Nucleation and Growth (Cham: Springer), 227–256. doi:10.1007/978-3-319-45669-0_12
Vass, A., Koehn, D., Toussaint, R., Ghani, I., and Piazolo, S. (2014). The Importance of Fracture-Healing on the Deformation of Fluid-Filled Layered Systems. J. Struct. Geol. 67, 94–106. doi:10.1016/j.jsg.2014.07.007
Vilarrasa, V., and Carrera, J. (2015). Geologic Carbon Storage Is Unlikely to Trigger Large Earthquakes and Reactivate Faults through Which CO 2 Could Leak. Proc. Natl. Acad. Sci. U.S.A. 112 (19), 5938–5943. doi:10.1073/pnas.1413284112
Vilarrasa, V., Rinaldi, A. P., and Rutqvist, J. (2017). Long-term Thermal Effects on Injectivity Evolution during CO2 Storage. Int. J. Greenh. Gas Control 64, 314–322. doi:10.1016/j.ijggc.2017.07.019
Vilarrasa, V., and Rutqvist, J. (2017). Thermal Effects on Geologic Carbon Storage. Earth-science Rev. 165, 245–256. doi:10.1016/j.earscirev.2016.12.011
Villar, M. V., and Lloret, A. (2004). Influence of Temperature on the Hydro-Mechanical Behaviour of a Compacted Bentonite. Appl. Clay Sci. 26 (1-4), 337–350. doi:10.1016/j.clay.2003.12.026
Virgo, S., Abe, S., and Urai, J. L. (2014). The Evolution of Crack Seal Vein and Fracture Networks in an Evolving Stress Field: Insights from Discrete Element Models of Fracture Sealing. J. Geophys. Res. Solid Earth 119 (12), 8708–8727. doi:10.1002/2014jb011520
IPCC (Forthcoming 2018). “Summary for Policymakers,” in Global Warming of 1.5°C. An IPCC Special Report on the Impacts of Global Warming of 1.5°C above Pre-industrial Levels and Related Global Greenhouse Gas Emission Pathways, in the Context of Strengthening the Global Response to the Threat of Climate Change, Sustainable Development, and Efforts to Eradicate Poverty. Editors V. Masson-Delmotte, P. Zhai, H. O. Pörtner, D. Roberts, J. Skea, P. R. Shuklaet al. Available at: https://www.ipcc.ch/site/assets/uploads/sites/2/2019/05/SR15_SPM_version_report_LR.pdf.
Wang, Q., Laubach, S. E., Gale, J. F. W., and Ramos, M. J. (2019). Quantified Fracture (Joint) Clustering in Archean Basement, Wyoming: Application of the Normalized Correlation Count Method. Pet. Geosci. 25 (4), 415–428. doi:10.1144/petgeo2018-146
Wang, X., Mao, X., Ji, L., Liu, H., Luo, L., Li, K., et al. (2019). “Karst Geothermal System in the Beijing-Tianjin-Hebei Plain of North China,” in PROCEEDINGS, 44th Workshop on Geothermal Reservoir Engineering Stanford University, Stanford, California, February 11-13, 2019.
Warpinski, N. R., and Teufel, L. W. (1987). Influence of Geologic Discontinuities on Hydraulic Fracture Propagation (Includes Associated Papers 17011 and 17074 ). J. Petroleum Technol. 39 (02), 209–220. doi:10.2118/13224-pa
Warren, J. K. (2006). Evaporites. Sediments, Resources and Hydrocarbons. Berlin, Heidelberg, New York: Springer-Verlag, XVI + 1035.
Warren, J. K. (2017). Salt Usually Seals, but Sometimes Leaks: Implications for Mine and Cavern Stabilities in the Short and Long Term. Earth-science Rev. 165, 302–341. doi:10.1016/j.earscirev.2016.11.008
Watson, M. N., Zwingmann, N., and Lemon, N. M. (2004). The Ladbroke Grove–Katnook Carbon Dioxide Natural Laboratory: a Recent CO2 Accumulation in a Lithic Sandstone Reservoir. Energy 29 (9–10), 1457–1466. doi:10.1016/j.energy.2004.03.079
Wersin, P., Spahiu, K., and Bruno, J. (1994). Time Evolution of Oxygen and Redox Conditions in HLW Repository. Stockholm: SKB TR 94-02.
White, C. M., Smith, D. H., Jones, K. L., Goodman, A. L., Jikich, S. A., LaCount, R. B., et al. (2005). Sequestration of Carbon Dioxide in Coal with Enhanced Coalbed Methane RecoveryA Review. Energy fuels. 19 (3), 659–724. doi:10.1021/ef040047w
Wiederhorn, S. M. (1967). Influence of Water Vapor on Crack Propagation in Soda-Lime Glass. J Am. Ceram. Soc. 50, 407–414. doi:10.1111/j.1151-2916.1967.tb15145.x
Wilkinson, M., Haszeldine, R. S., Fallick, A. E., Odling, N., Stoker, S. J., and Gatliff, R. W. (2009). CO2-Mineral Reaction in a Natural Analogue for CO2 Storage--Implications for Modeling. J. Sediment. Res. 79, 486–494. doi:10.2110/jsr.2009.052
Williams, J. D. O., Fellgett, M. W., and Quinn, M. F. (2016). Carbon Dioxide Storage in the Captain Sandstone Aquifer: Determination of In Situ Stresses and Fault-Stability Analysis. Pet. Geosci. 22, 211–222. doi:10.1144/petgeo2016-036
Wilson, L., Wilson, M. J., Green, J., and Patey, I. (2014). The Influence of Clay Mineralogy on Formation Damage in North Sea Reservoir Sandstones: a Review with Illustrative Examples. Earth-Science Rev. 134, 70–80. doi:10.1016/j.earscirev.2014.03.005
W. Miller, R. Alexander, N. Chapman, J. C. McKinley, and J. A. T. Smellie (Editors) (2000). Geological Disposal of Radioactive Wastes and Natural Analogues (Elsevier), 2.
Wong, L. N. Y., Maruvanchery, V., and Liu, G. (2016). Water Effects on Rock Strength and Stiffness Degradation. Acta Geotech. 11, 713–737. doi:10.1007/s11440-015-0407-7
Wood, J. R. (1986). Thermal Mass Transfer in Systems Containing Quartz and Calcite. McLean, Virginia, US: GeoScienceWorld.
Wu, Y., and Li, P. (2020). The Potential of Coupled Carbon Storage and Geothermal Extraction in a CO 2-enhanced Geothermal System: a Review. Geotherm. Energy 8 (1), 1–28. doi:10.1186/s40517-020-00173-w
Yamamoto, H., and Kennedy, G. C. (1969). Stability Relations in the System CaSO4-H2o at High Temperatures and Pressures. Am. J. Sci. 267A, 550–557.
Yanagisawa, N. (2015). “Case Study of Calcium Carbonate Scale at EGS and Hot Spring Binary System,” in Proceedings World Geothermal Congress, Melbourne, Australia, 19-25 April 2015.
Yang, Z., Xia, G. G., Walker, M. S., Wang, C. M., Stevenson, J. W., and Singh, P. (2007). High Temperature Oxidation/Corrosion Behavior of Metals and Alloys Under a Hydrogen Gradient. Int. J. Hydrog. Energy 32 (16), 3770–3777. doi:10.1016/j.ijhydene.2006.08.056
Yoon, J. S., Zang, A., and Stephansson, O. (2013). “Hydro-mechanical Coupled Discrete Element Modeling of Geothermal Reservoir Stimulation and Induced Seismicity,” in Clean Energy Systems in the Subsurface: Production, Storage and Conversion (Berlin, Heidelberg: Springer), 221–231. doi:10.1007/978-3-642-37849-2_19
Yoshida, H., Aoki, K., Semba, T., Ota, K., Amano, K., Hama, K., et al. (2000). Overview of the Stability and Barrier Functions of the Granitic Geosphere at the Kamaishi Mine: Relevance to Radioactive Waste Disposal in Japan. Dev. geotechnical Eng. 84, 159–170. doi:10.1016/s0165-1250(00)80014-0
You, D., Han, J., Hu, W., Qian, Y., Chen, Q., Xi, B., et al. (2018). Characteristics and Formation Mechanisms of Silicified Carbonate Reservoirs in Well SN4 of the Tarim Basin. Energy Explor. Exploitation 36 (4), 820–849. doi:10.1177/0144598718757515
Younger, P. L., and Adams, R. (1999). Predicting Mine Water Rebound. W179. Newcastle upon Tyne, England: Newcastle University.
Younger, P. L. (1995). Hydrogeochemistry of Minewaters Flowing from Abandoned Coal Workings in County Durham. Q. J. Eng. Geol. Hydrogeology 28 (Suppl. 2), S101–S113. doi:10.1144/GSL.QJEGH.1995.028.S2.02
Zeng, W., Zhang, J., Ding, W., Zhao, S., Zhang, Y., Liu, Z., et al. (2013). Fracture Development in Paleozoic Shale of Chongqing Area (South China). Part One: Fracture Characteristics and Comparative Analysis of Main Controlling Factors. J. Asian Earth Sci. 75, 251–266. doi:10.1016/j.jseaes.2013.07.014
Zhang, C., Pathegama Gamage, R., Perera, M., and Zhao, J. (2017). Characteristics of Clay-Abundant Shale Formations: Use of CO2 for Production Enhancement. Energies 10 (11), 1887. doi:10.3390/en10111887
Zhang, D., and Song, J. (2014). Mechanisms for Geological Carbon Sequestration. Procedia IUTAm 10, 319–327. doi:10.1016/j.piutam.2014.01.027
Zhu, D., Jin, Z., and Hu, W. (2010). Hydrothermal Recrystallization of the Lower Ordovician Dolomite and its Significance to Reservoir in Northern Tarim Basin. Sci. China Earth Sci. 53 (3), 368–381. doi:10.1007/s11430-010-0028-9
Zhu, D., Jing, H., Yin, Q., and Han, G. (2018). Experimental Study on the Damage of Granite by Acoustic Emission after Cyclic Heating and Cooling with Circulating Water. Processes 6 (8), 101. doi:10.3390/pr6080101
Zidane, A., Zechner, E., Huggenberger, P., and Younes, A. (2014). On the Effects of Subsurface Parameters on Evaporite Dissolution (Switzerland). J. Contam. hydrology 160, 42–52. doi:10.1016/j.jconhyd.2014.02.006
Zimmermann, G., Tischner, T., Legarth, B., and Huenges, E. (2009). Pressure-dependent Production Efficiency of an Enhanced Geothermal System (EGS): Stimulation Results and Implications for Hydraulic Fracture Treatments. Pure Appl. Geophys. 166, 1089–1106. doi:10.1007/s00024-009-0482-5
Keywords: thermal-hydrological-mechanical-chemical (THMC) processes, geological strata, geothermal energy, nuclear waste disposal, CCS
Citation: Kaminskaite I, Piazolo S, Emery AR, Shaw N and Fisher QJ (2022) The Importance of Physiochemical Processes in Decarbonisation Technology Applications Utilizing the Subsurface: A Review. Earth Sci. Syst. Soc. 2:10043. doi: 10.3389/esss.2022.10043
Received: 28 June 2021; Accepted: 18 May 2022;
Published: 20 June 2022.
Edited by:
Christopher M. Yeomans, University of Exeter, United KingdomReviewed by:
Stephen Laubach, University of Texas at Austin, United StatesAndreas Busch, Heriot-Watt University, United Kingdom
Copyright © 2022 Kaminskaite, Piazolo, Emery, Shaw and Fisher. This is an open-access article distributed under the terms of the Creative Commons Attribution License (CC BY). The use, distribution or reproduction in other forums is permitted, provided the original author(s) and the copyright owner(s) are credited and that the original publication in this journal is cited, in accordance with accepted academic practice. No use, distribution or reproduction is permitted which does not comply with these terms.
*Correspondence: Ieva Kaminskaite, ieva.kaminskaite888@gmail.com
†Present address: Ieva Kaminskaite, School of Earth Science, Northeast Petroleum University, Daqing, China