- 1British Geological Survey, Edinburgh, United Kingdom
- 2British Geological Survey, Nottingham, United Kingdom
- 3Scottish Universities Environmental Research Centre, East Kilbride, United Kingdom
- 4Civil and Environmental Engineering, University of Strathclyde, Glasgow, United Kingdom
- 5Grant Institute, University of Edinburgh, Edinburgh, United Kingdom
- 6The Lyell Centre, Heriot-Watt University, Edinburgh, United Kingdom
- 7School of Geographical and Earth Sciences, University of Glasgow, Glasgow, United Kingdom
- 8Camborne School of Mines and Environment and Sustainability Institute, University of Exeter Penryn, Cornwall, United Kingdom
Mine water geothermal energy could provide sustainable heating, cooling and storage to assist in the decarbonisation of heat and achieving Net Zero carbon emissions. However, mined environments are highly complex and we currently lack the understanding to confidently enable a widespread, cost-effective deployment of the technology. Extensive and repeated use of the mined subsurface as a thermal source/store and the optimisation of operational infrastructure encompasses a range of scientific and technical challenges that require broad partnerships to address. We present emerging results of a pioneering multidisciplinary collaboration formed around an at-scale mine water geothermal research infrastructure in Glasgow, United Kingdom. Focused on a mined, urban environment, a range of approaches have been applied to both characterise the environmental change before geothermal activities to generate “time zero” datasets, and to develop novel monitoring tools for cost-effective and environmentally-sound geothermal operations. Time zero soil chemistry, ground gas, surface water and groundwater characterisation, together with ground motion and seismic monitoring, document ongoing seasonal and temporal variability that can be considered typical of a post-industrial, urban environment underlain by abandoned, flooded coal mine workings. In addition, over 550 water, rock and gas samples collected during borehole drilling and testing underwent diverse geochemical, isotopic and microbiological analysis. Initial results indicate a connected subsurface with modern groundwater, and resolve distinctive chemical, organic carbon and stable isotope signatures from different horizons that offer promise as a basis for monitoring methods. Biogeochemical interactions of sulphur, carbon and iron, plus indications of microbially-mediated mineral oxidation/reduction reactions require further investigation for long term operation. Integration of the wide array of time zero observations and understanding of coupled subsurface processes has significant potential to inform development of efficient and resilient geothermal infrastructure and to inform the design of fit-for-purpose monitoring approaches in the quest towards meeting Net Zero targets.
Introduction
Central to achieving Net Zero carbon emissions targets in the UK will be the decarbonisation of heating and cooling of our buildings (HM Government, 2020). In 2020, 90% of UK homes used fossil fuels (predominantly gas) for heating, cooking and hot water (HM Government, 2020) with UK domestic heat demand between 300 and 400 TWh/yr between 2003 and 2015 (Watson et al., 2019). Globally, heating and cooling accounted for 51% of energy demand in 2018, with 10.2% met from renewable energy (Ren21, 2021), though the picture is varied with the “fuel share” for heating demand of countries such as Sweden and Norway being less than 10% for gas, oil and coal (Gross and Hanna, 2019). To date, progress to decarbonise heat in the UK has been slow due to factors such as paucity of heat networks, retrofitting of existing buildings, lack of subsurface heat regulation (Abesser, 2020; HM Government, 2020; Postnote, 2020) and lack of strong policy signalling (e.g., Committee on Climate Change, 2016; Gross and Hanna, 2019). The sector is rapidly evolving with the emergence of growing numbers of policy/strategy announcements and financial schemes [e.g., UK Government Green Heat Network Fund, Net Zero Strategy (HM Government, 2021), Scottish Government Heat in Buildings Strategy and Scottish Heat Network Fund], including wider recognition that geothermal energy could form an important part of decarbonisation of heat (e.g., Lund and Toth, 2021; REA/Arup 2021; Abesser and Walker, 2022).
Using the shallow underground (≤500 m) for heating, cooling and thermal storage offers a potentially sustainable low carbon solution for the energy transition. Mine water geothermal utilises the warm water in abandoned, flooded coal mines that are widespread beneath many of the UK’s towns and cities (Bailey et al., 2016; Farr et al., 2020; Walls et al., 2021). This anthropogenic aquifer has the potential to supply renewable heat to homes and businesses coincident with the coalfields, which are commonly locations of heat demand. Less than fifty documented operating schemes in abandoned coal mines have proved the concept of mine water heating, cooling and inter-seasonal storage globally including in Germany, Spain, Netherlands, Poland, United States, Russia and China, with the UK currently having around 2.6 MWth of mine water heat installed and 9 MWth currently under advanced exploration or construction stages (e.g., Verhoeven et al., 2014; Banks et al., 2017; Loredo et al., 2017; Adams et al., 2019; The Coal Authority, 2020; Busby and Mansor, 2021; Steven, 2021; Walls et al., 2021). Estimates of the mine water thermal resource are large, for example 2.7 TWh/yr from the Midland Valley of Scotland heat-in-place (not the recoverable resource), equivalent to 8% of Scotland’s annual domestic heating demand (Todd et al., 2019), or c. 16 TWh of potential UK underground thermal storage (Gluyas et al., 2020, ΔT 5°C scenario) equivalent to around 5% of annual UK domestic heat demand (Watson et al., 2019). The potential in other countries with abandoned, flooded coal mines is also significant (e.g., US Watzlaf and Ackman, 2006; Germany Bracke and Bussmann, 2015; generally Preene and Younger, 2014; Ramos et al., 2015).
To enable the widespread rollout of mine water heat, the costs and technical risks need to be significantly reduced along with changes to policy, regulation and financial incentives (NERC, University of Strathclyde and BGS, 2019; Optimat, 2019; NELEP, 2021; Townsend et al., 2021). The technical aspects of subsurface exploration, sustainable operation and heat distribution spans a wide range of disciplines: geoscience, engineering, biochemistry, economics, and social science. Consequently, geoscientists are increasingly aware of their role in the whole energy chain. For example, the social acceptability for onshore geoenergy technologies relies on risks and uncertainties being better understood, community-focused engagement and energy framing (Dickie et al., 2020; Demski, 2021). Increasing the geoscientific evidence base through new scientific data is at the core of this energy chain (Figure 1). For mine water geothermal, one such research area that we know relatively little about is the consequences of chemical, biological and physical processes acting in an anthropogenically-altered underground, how these are impacted by the repeated cycles of heat and water flow of geothermal operations, and by competing uses of the subsurface for resources, such as water supply, and infrastructure.
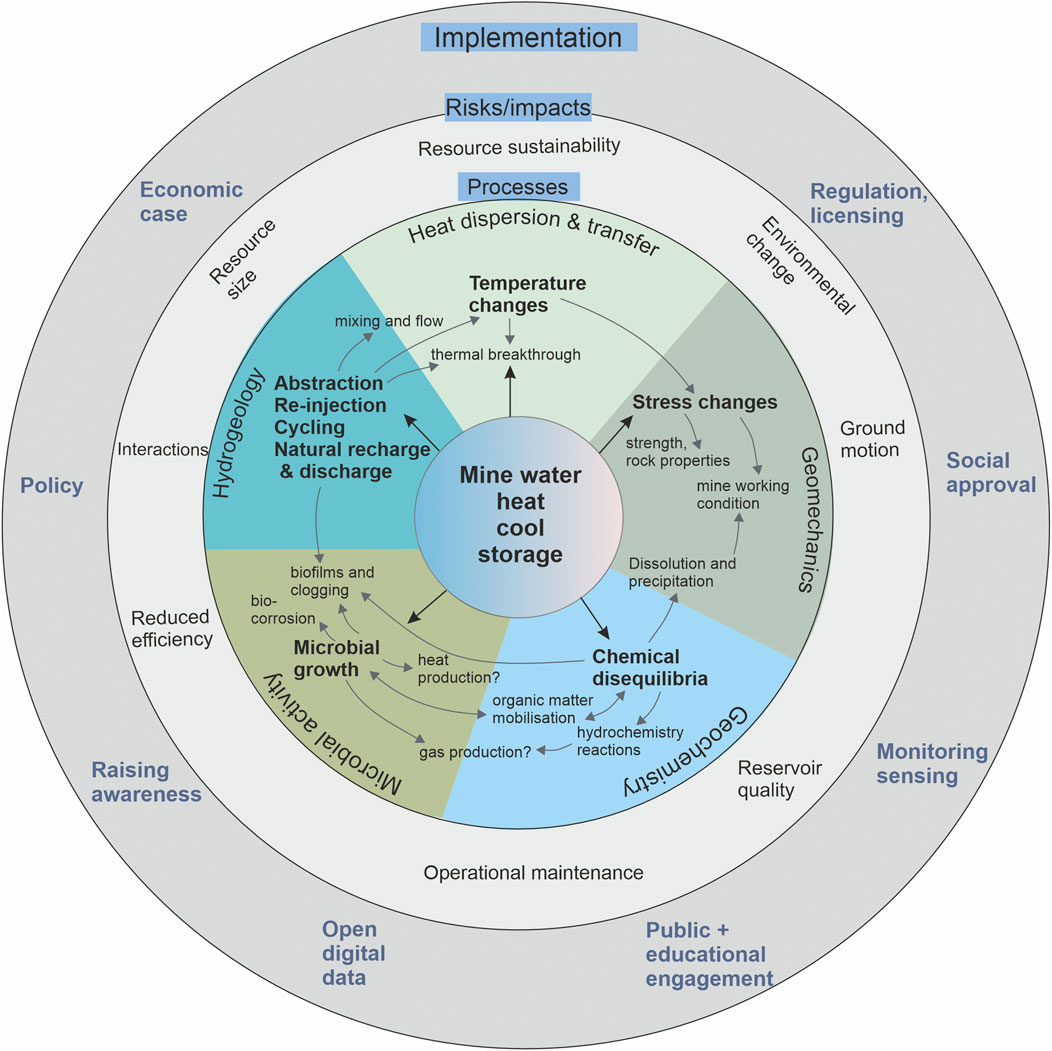
FIGURE 1. Summary of key geoscientific subsurface processes in mine water heating, cooling or storage (core of figure), resultant potential risks and impacts (second ring), mapped outwards to implementation/outcomes/benefits (outer ring).
Real-world quantitative evidence of coupled rock and fluid processes, ongoing and induced environmental change for de-risking can happen at multidisciplinary, at-scale test sites (Jenkins et al., 2012; Stephenson et al., 2019; Figure 1 outer ring). The UK Geoenergy Observatory in Glasgow is one such example, specifically designed around mine water heat (Monaghan et al., 2021a).
The UK Geoenergy Observatory (hereafter referred to as the Glasgow Observatory, or simply Observatory) is located in the south-east of Glasgow city region with the majority of the infrastructure situated within the Cuningar Loop, Rutherglen (Figure 2). It is a unique facility for monitoring, testing and innovation focused on process understanding of mine water heat, funded by UK Government through UK Research and Innovation/Natural Environment Research Council, delivered and operated by the British Geological Survey (BGS). The planning, construction and testing of the boreholes was delivered between 2016 and 2020 and the Observatory is planned to have a 15-year operational lifespan (Monaghan et al., 2021a; Starcher et al., 2021). It comprises 12 boreholes across five sites–five groundwater environmental monitoring boreholes (16–45 m mbgl), five mine water characterisation and monitoring boreholes screened at the Glasgow Upper mine working (c. 50 m mbgl) or Glasgow Main mine working (c. 85 m mbgl), a sixth borehole repurposed for sensor testing to 67 m depth, and a 199 m deep seismic monitoring borehole that produced the cored reference section (Figures 2, 3). Downhole electrical resistivity tomography sensors, fibre-optic cables for distributed temperature sensing and hydrogeological data loggers enable time-series monitoring to characterise physical, chemical and flow heterogeneities. Permanent geothermal “sealed open loop” infrastructure for research into the abstraction and re-injection of mine water and extraction or storage of heat is being installed on four of the existing mine water boreholes for completion in mid-2022. The 200 kW output is at the scale of a small mine water heat scheme, such as may supply a municipal building; for example in Essen, Germany a scheme of comparable size was used to heat a retirement complex (Hall et al., 2011). With far greater monitoring than would be expected of a commercial geothermal scheme, the extensive data gathered at the Glasgow Observatory on processes, developing monitoring tools etc. has wide applicability to similar settings, and to regulatory and policy development.
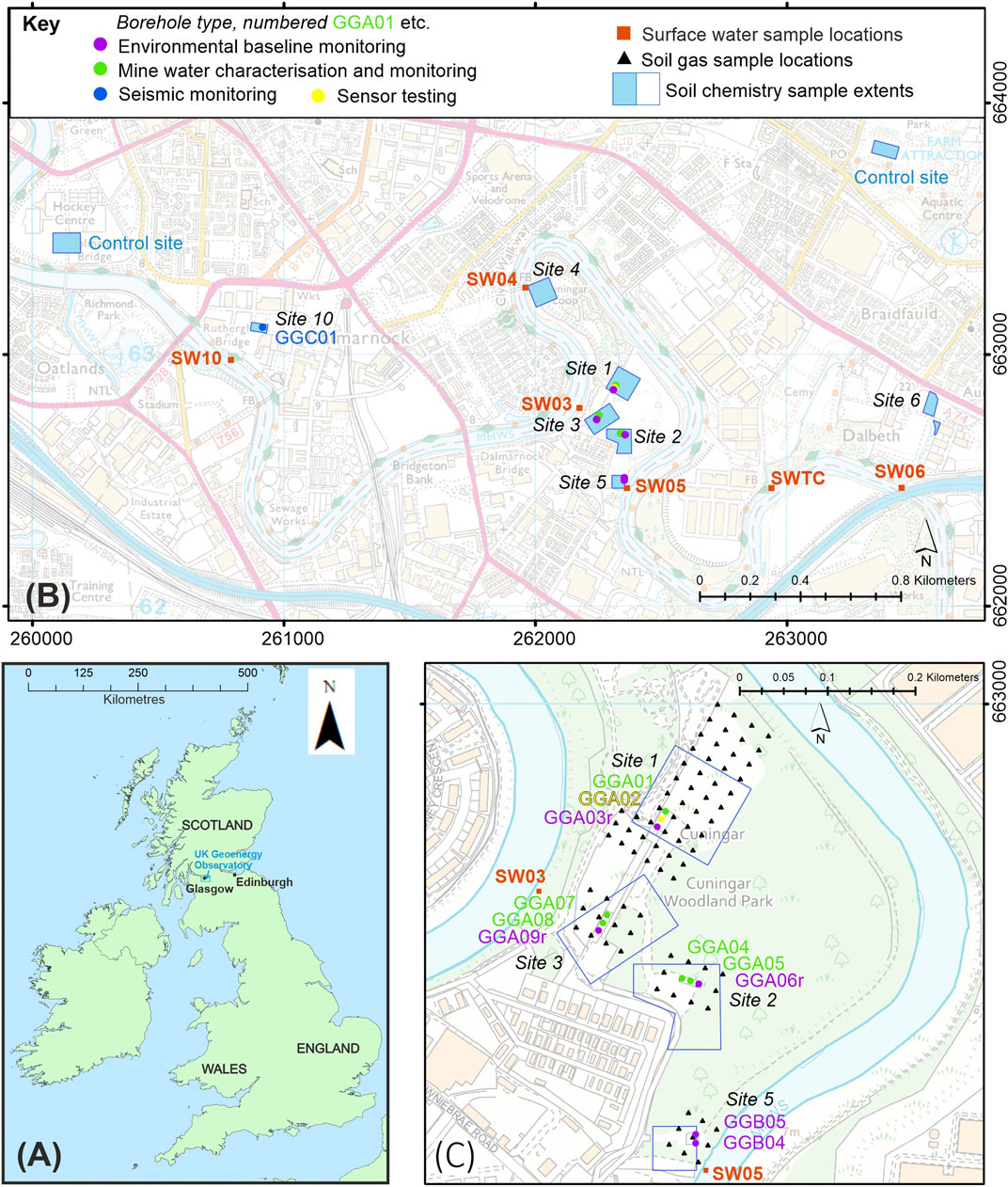
FIGURE 2. Location maps (A) location of the UK Geoenergy Observatory (B) overview of surface water, soil chemistry and borehole sites in Glasgow city region (C) detail of boreholes and ground (soil) gas survey points at Cuningar Loop. Ordnance Survey data ©Crown Copyright and database rights 2021. Ordnance Survey Licence No. 100021290 EUL.
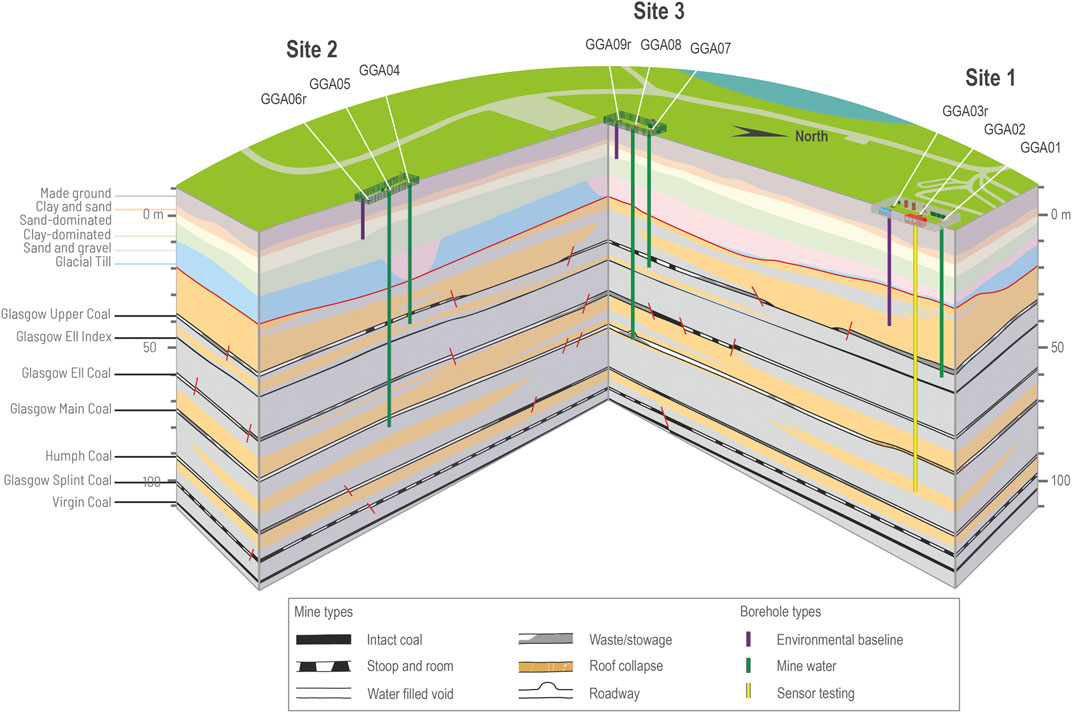
FIGURE 3. Interpretive 3D block diagram to illustrate borehole geometry, proved and interpreted mine working variability indicated by abandonment plans at the UK Geoenergy Observatory in Glasgow. No vertical exaggeration. Height in metres relative to Ordnance Datum shown (ground level around 10–12 m above OD) Reproduced from Monaghan et al. (2021a), BGS © UKRI 2021.
This paper highlights some of the first time-series datasets that form the environmental baseline characterisation at the shallow mine water geothermal observatory at “time zero”—before construction, during construction and through borehole testing. We highlight that by multidisciplinary working we can uniquely explore coupled hydrogeological-chemical-biological processes and use these to provide an innovative insight to future research challenges in this complex environment (Figure 1; inner rings). This characterisation and monitoring forms the basis to evaluate any future changes induced by geothermal heat extraction and flow cycling. It informs the resource size and sustainability, environmental impact and may contribute towards social approval of mine water heat, towards innovation of new technologies and reducing cost and risk for future heat supply schemes.
We describe a novel multidisciplinary and multi-institution approach to integrative applied geoscience required to address current societal need, unusual in the terrestrial environment outside of large grant funding. The approach initiated from during drilling and testing samples provided to five Universities during construction of the Glasgow Observatory. Open access data and collaborative discussions to maximise understanding have enabled a subsurface systems approach, more diverse and explorative than may have been achieved without integration, illustrating the power of providing scientific access to such sites prior to, and during, the construction phase.
Method and Materials: During Drilling and Baseline Sampling Campaigns
In and around the Glasgow Observatory, a suite of environmental baseline characterisation and monitoring data has been collected by the site operator (BGS) on the physical properties of the aquifer, the chemical properties of groundwater and surface water, the land quality (soil geochemistry), ground gas measurement, together with seismicity and ground movement over larger areas (Figure 2; Bateson and Novellino, 2019; Barkwith et al., 2020; Fordyce et al., 2020a; Fordyce et al., 2021; Palumbo-Roe et al., 2021; Shorter et al., 2021a,b). The environmental baseline characterises the prevailing natural and anthropogenic conditions; this paper includes baseline measurements and surveys from 2018 to 2020.
In addition, opportunities were advertised by BGS for researchers to request samples before two periods of borehole drilling commenced. This early access “during drilling” sampling covered time-dependent core sampling, rock chip samples, drilling fluid and groundwater. This paper highlights some initial results of 149 core, 120 rock chip, 217 during-construction drilling fluid/groundwater and 66 post-drilling groundwater samples that were collected and distributed to 12 academic researchers in 5 universities, covering research on geochemistry, isotopic characterisation, gases, organic carbon and geomicrobiology (Figure 2; Table 1). A detailed Supplementary Table S1 is provided in supplementary information that details the purpose and timing of sampling for each research group. The detailed methodologies, results and data are available in open data packs for baseline characterisation and monitoring via the National Geoscience Data Centre or links on the ukgeos.ac.uk website.
Baseline surveys and sampling were undertaken before drilling, during drilling, during borehole cleaning and test pumping (Figure 4), providing the time zero baseline through the exploration stages of a mine water geothermal infrastructure. Samples represent both the natural environment (e.g., rock chips, surface water and soil) plus responses to, and monitoring of, drilling into the subsurface environment (e.g., borehole drilling return fluids, settling tank fluids). Analysis and reporting presented here are categorised through drilling stages, borehole depth (time-series) and lithologically.
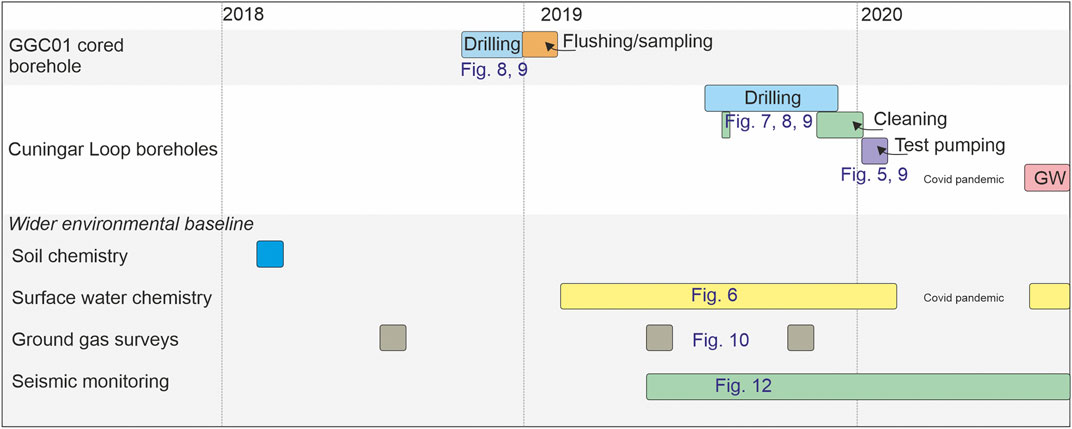
FIGURE 4. Overview of sample, surveys and monitoring through time during construction of the UK Geoenergy Observatory in Glasgow. Boreholes progressed through drilling, cleaning, test pumping and groundwater chemistry sampling (GW). Blue numbering shows corresponding result figures in this paper.
Stakeholder engagement, including with the public, was a critical part of the development of the Glasgow Observatory, running in parallel to the time zero baseline sampling and characterisation described here. Public engagement events were held with the local community, enabling open dialogue with local residents, resulting in the repositioning of some boreholes, and establishing a relationship that continued throughout the drilling and construction process. Community engagement identified that potential or perceived environmental impacts from construction and geothermal activities were a concern, further justifying the need for an environmental baseline. A separate study found that awareness of geothermal technologies was low (Dickie et al., 2020).
Borehole Drilling and Testing
Here, we present the method, results and initial interpretation for different studied parameters in turn, giving information on the context and rationale as we do so.
Geology and Mine Water Reservoir
The superficial deposits across the Glasgow Observatory range from 26 to 40 m in thickness, including 7.5–9 m of made ground dominated by materials consistent with building demolition landfill. The natural Quaternary succession is typical of that in the River Clyde valley and eastern Glasgow, comprising glacial till and marine, lacustrine and fluvio-glacial deposits, overlain by fluvial deposits (Browne and McMillan, 1989; Arkley and Callaghan, 2021). At Cuningar Loop, the superficial deposits beneath the fluvial deposits and raised marine estuarine clays proved to be more sand- and gravel-dominated than predicted, with channelised glacio-fluvial deposits interpreted as cutting into glacial till (Arkley and Callaghan, 2021; Monaghan et al., 2021a; Figure 3).
The bedrock succession in this part of eastern Glasgow comprises gently folded Carboniferous sedimentary strata that are cut by faults of metres to hundreds of metres throw, on a variety of orientations. Nine observatory boreholes penetrated bedrock typical of the Scottish Coal Measures Group, with interbedded mudstone, siltstone, sandstone and coal (Figure 3; Table 2). Borehole GGC01 at Dalmarnock provided a c.170 m cored section containing 9 intact coal seams (Monaghan et al., 2021b). At Cuningar Loop, mine water boreholes encountered workings in the Glasgow Upper, Glasgow Ell and Glasgow Main coal seams, proving water-filled voids, mine waste, fractured rock mass and intact coal pillars (Monaghan et al., 2021a; Table 2). Three boreholes are screened across the Glasgow Upper mine working (GGA01, GGA04, GGA07) and two screened across the Glasgow Main mine working (GGA05, GGA08; Figure 3). These are representative of different mining styles and post-abandonment history with groundwater flow likely to be via combination of pipe flow (voids), porous media (mine “wastes” (material packed into workings, or roof collapse into workings), surrounding sandstones) and fracture flow.
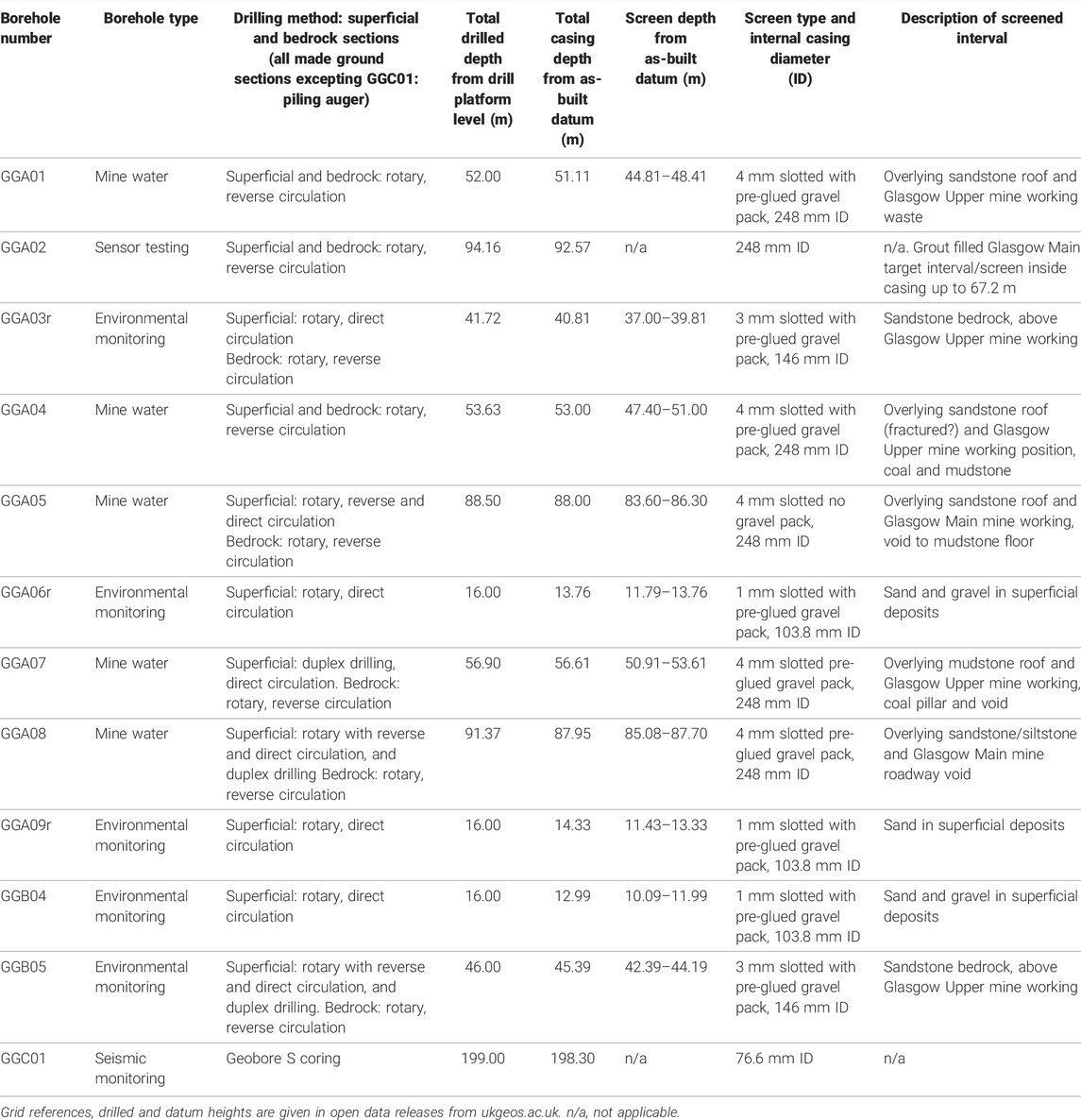
TABLE 2. Summary of boreholes at the Glasgow Observatory: drilling type, internal casing diameter and screened horizon.
The successions are typical of many coalfield areas where the post-industrial legacy includes a complex and anthropogenically-altered groundwater and surface water hydrogeological and hydrochemical environment comprising not only coal-mine waters, mine working roadways, shafts and discharges, but also a top layer of made ground that may include contaminants of concern.
Hydrogeology and Groundwater Chemistry
Initial hydrogeological indications were gained throughout borehole drilling, with sample analysis from the drilling flush, settling tank, borehole water and borehole cleaning stages presented in separate sections below. A small number of standard geochemistry analyses taken by BGS during borehole construction (Shorter et al., 2021b) were followed by the results from test pumping that represent purged samples of groundwater from the aquifer unit.
Characterisation of the hydrogeological system and viable yields from abstraction boreholes are critical to evaluating mine water heat resources and understanding coupled systems and processes in the rock-water-biosphere environment. In addition, these data inform environmental protection. As a first step, pumping tests were carried out by BGS in ten of the boreholes at the Glasgow Observatory to: determine the physical aquifer properties of the mine workings, bedrock and superficial deposits; test the yield of the boreholes; identify connectivity between units; and to take pumped samples for water chemistry characterisation and residence time analysis. Step tests and five-hour constant rate tests were undertaken at nine boreholes, whilst a falling and rising head test was carried out on the low yielding borehole GGB04 (Shorter et al., 2021a; Figure 2 and Table 3). Water levels were monitored in pumped and observation boreholes (Figure 5) and preliminary transmissivity measurements estimated using Jacob’s approximation and the Theis recovery method (Table 3). Not all assumptions were wholly met for analysis using the Jacob’s Method or Theis recovery which assume homogeneous isotropic conditions with intergranular flow. However, research and modelling has shown that transmissivity estimated using these methods in more heterogeneous aquifers can still give realistic results (Sanchez-Vila et al., 1999; Halford et al., 2006). Samples were taken during the tests for major ions, trace elements and a range of organic carbon compounds, dissolved gases, and stable isotopes (δ2H, δ18O, δ13C, δ34S; Table 1 BGS analyses; Palumbo-Roe et al., 2021).
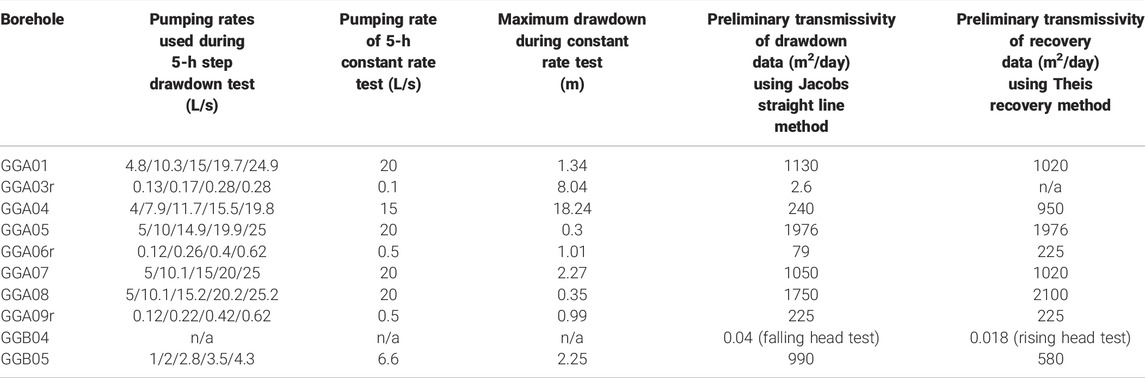
TABLE 3. Summary of initial test pumping of nine boreholes, plus slug test on one borehole, at the Glasgow Observatory, data summarised from Shorter et al. (2021).
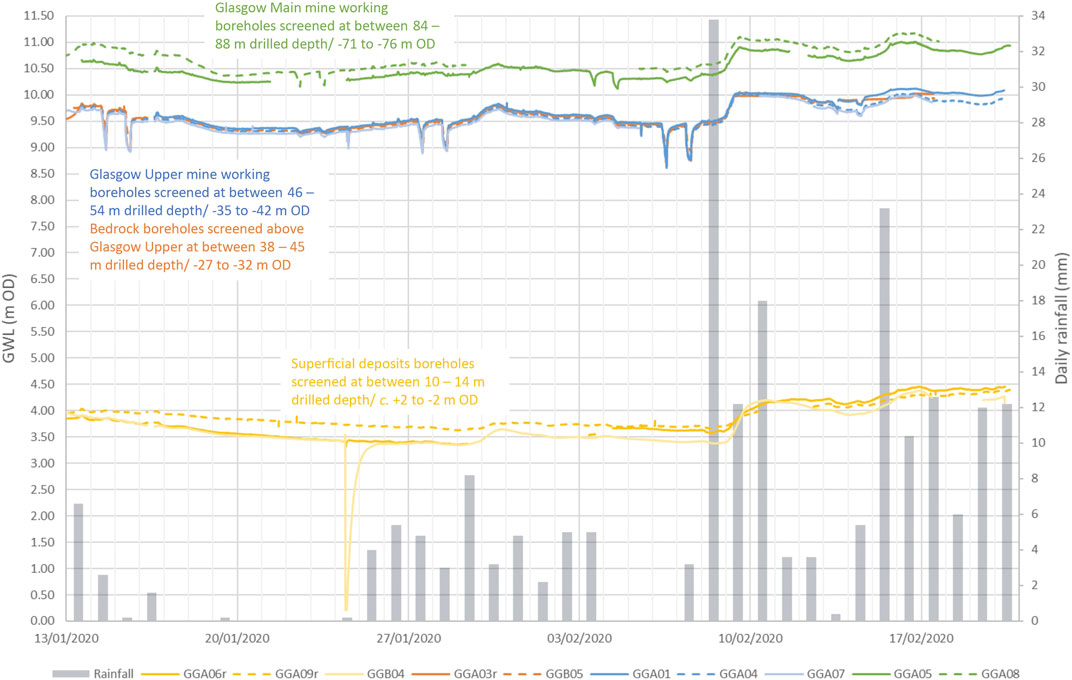
FIGURE 5. Overview of groundwater levels (GWL) in ten boreholes in metres relative to Ordnance Datum (OD) during the pumping test period January–February 2020. In grey, SEPA rainfall data © Scottish Environment Protection Agency and database right 2021. All rights reserved. Glasgow Main mine working boreholes in green, Glasgow Upper mine working boreholes in blue, bedrock boreholes in orange, superficial deposit boreholes in yellow, showing that piezometric head levels are highest in the Glasgow Main mine working, distinctly different levels in the superficial deposits, plus the response to high rainfall. Sharp spikes are test pumping periods.
The test pumping gave maximum drawdowns of 0.3–18 m across the test sites. The transmissivity of the superficial deposits at the Observatory are highly variable (<1 to >200 m2/day), reflecting the diversity of superficial material, but consistent with previously measured transmissivity values in Glasgow (Williams, et al., 2017). The test pumping responses in the two bedrock boreholes gave very different results, likely reflecting the importance of fracturing within the sandstone, including fracturing induced in the overlying bedrock by mining. The transmissivity was similar within mine workings - approximately 1000 m2/day for the three tests in the Glasgow Upper and approximately 2000 m2/day for the two tests in the Glasgow Main—despite the boreholes penetrating mine workings of diverse character from open voids, to waste and likely fractured coal, and likely incorporating a range of local hydrogeological conditions. Data on transmissivity are rare for Coal Measures strata (Jones et al., 2000; Graham et al., 2009), but the results are at the higher end of estimates from available Scottish pumping test data (Ó Dochartaigh et al., 2015). The temperatures measured during the pumping tests were in the range 11.5–12.5°C, with the highest temperatures observed in the deeper Glasgow Main mine working.
Characterising the chemistry of the groundwaters from test pumping sampling reveals that all the groundwaters are moderately mineralised (1500–2000 μS/cm), with near neutral pH, and comprise bicarbonate–type waters. They contain sufficient alkalinity (HCO3 range 731–943 mg/L) to neutralise the mineral acidity, and are therefore classed as net alkaline mine waters, in common with many of the flooded mine workings in Scotland (Younger, 2001). The carbon isotope signature δ13C of dissolved inorganic carbon (DIC), averages −10.9‰. Anoxic conditions are common to all the groundwaters, with dissolved oxygen <0.5 mg/L; nitrate concentrations <0.6 mg/L, and high concentrations of dissolved iron (range 417–19,500 μg/L), manganese (range 260–3100 μg/L) and ammonium (up to 23 mg/L). Most inorganic parameters are within the range of groundwater measured from mined Carboniferous rocks in Scotland (MacDonald et al., 2017). Multivariate statistical cluster analysis indicates that the superficial deposits, bedrock groundwaters and the mine-workings are each clustered into statistically distinct groups based on their chemical composition (Palumbo-Roe et al., 2021). Groundwater stable isotope ratios (δ2H and δ18O) show a general correspondence with the global meteoric water line indicating that the groundwaters represent recharge from local rainfall consistent with the current climate. Interpretation of residence time data from CFC-11, CFC-12 and SF6 suggests that the average mean residence time of the groundwater is between 50 and 70 years in all the aquifer units. The youngest waters occur in the deepest boreholes installed within the Glasgow Main mine workings.
Groundwater level monitoring consistently shows that the piezometric surface in the deeper mine workings is higher than the shallower workings and both are higher than in the superficial deposits (Figure 5), consistent with the site being a discharge zone. In all boreholes, water levels respond to rainfall, the most notable example after a large rainfall event in February 2020 (Figure 5). The initial interpretation of the pumping tests and chemistry indicate strong connectivity within the individual mine workings and strong connections between the overlying sandstone bedrock and the adjacent Glasgow Upper mine working (orange and blue lines Figure 5). There is evidence of connectivity between the two mine workings, but little evidence of direct connectivity with the superficial deposits. Synthesis of these data towards an initial hydrogeological conceptual model is discussed below.
Surface Water Chemistry
Prior to and during borehole construction and testing, monitoring of the chemical quality of surface water was carried out between February 2019 and March 2020. The monitoring has established the surface water temporal and spatial chemical variability against which future change can be assessed and contributes to the characterisation of the hydrological/hydrogeological system in the area. Monthly surface water sampling was carried out at six sampling locations for inorganic, organic and isotopic analyses (Table 1) in the lower reaches of the River Clyde catchment. The River Clyde is a major water body with a catchment of about 2000 km2, flowing from east to west through urban Glasgow with mean annual flow rate at 48 m3/s (at Daldowie [NS 67154 61642], National River Flow Archive, 2022), fed by numerous tributaries for which regional stream sediment and surface water geochemical surveys have been conducted (Fordyce et al., 2004). On the River Clyde, three locations were monitored proximal to the Observatory at Cuningar Loop and two control sites approximately 1.5 km upstream and 2 km downstream (Figure 2B); all upstream of the weir that limits the tidal extent of the river. An additional monitoring point was at Tollcross Burn (SWTC in Figure 2B), a small tributary of the River Clyde (Fordyce et al., 2021).
The surface waters are primarily circum-neutral to alkaline pH (7.4–8.2) calcium-bicarbonate (Ca-HCO3) waters, likely reflecting the calcareous nature of the underlying geological parent materials and presence of anthropogenic carbonate-rich materials, such as building rubble, in the urban environment of Glasgow and Rutherglen. Across the monitoring zone, the River Clyde shows little spatial variability in the majority of the parameters, except for minor differences which may reflect greater influence of local bank seepage and poor mixing at certain locations and times. The surface water chemistry dataset showed significant temporal variability, related to seasonal/climatic patterns, rainfall and contaminant inputs. Parameters exhibiting seasonality include dissolved organic carbon (3.0–17.3 mg/L), temperature (3.6–21.9°C) and stable isotope data (Fordyce et al., 2021). Major ion concentrations were higher in the River Clyde during periods of lower rainfall (April–June 2019), when baseflow was likely more dominant. Several trace elements show similar temporal behaviour in the River Clyde including arsenic, antimony, barium, caesium, cobalt, rubidium, strontium, selenium, tin and uranium (Figure 6: iron).
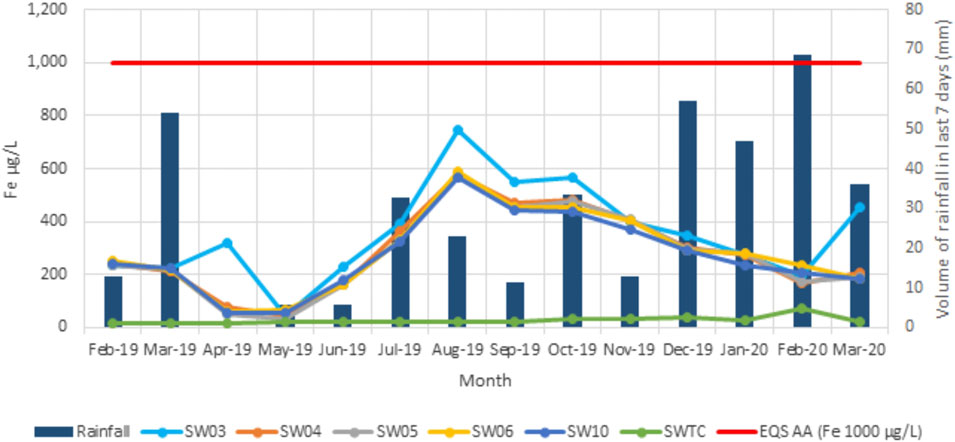
FIGURE 6. An example time-series plot of surface water chemistry: iron concentrations. Each sampling location (Figure 2B) is shown in a different colour. Contains SEPA rainfall data © Scottish Environment Protection Agency and database right 2021. All rights reserved. EQS AA = environmental quality standard annual average for good river status (UKTAG, 2013; SEPA, 2014; SEPA, 2019).
The range of δ13C values is typical for surface waters (δ13C −10.5 to −25.6‰). The δ2H and δ18O data are within ranges reported previously for surface waters in the west of Scotland (δ2H −66.9 to −39.3‰; δ18O−9.5 to −6.6‰; Darling et al., 2003; Tyler et al., 2016; Birkel et al., 2018). The δ2H and δ18O data plot slightly above the global meteoric water line (GMWL), but this is likely because waters in the west of Scotland are known to be more enriched, especially in δ2H, as a result of the predominance of moist-Atlantic weather fronts and higher rainfall. There is some evidence of seasonal control on the isotopic signatures, with more enriched δ2H (>−47‰) and δ18O (>−7.5‰) values reported in the summer months (June–September 2019; Fordyce et al., 2021).
For the majority of parameters, the concentrations recorded in 2019–2020 are within or similar to the ranges reported from the River Clyde and urban streams within the Glasgow area in 2002 and 2003 (Fordyce et al., 2004; Smedley et al., 2017). Regulatory authorities class the River Clyde in Glasgow as a highly modified water body with moderate ecological status. Initial comparisons with the good river environmental quality standard (EQS) annual average (AA) and maximum allowable concentrations (MAC; SEPA, 2014; SEPA, 2019; UKTAG, 2013) show that the majority of parameters are within these limits.
During Observatory borehole construction, test pumping and controlled discharges of waste water at SW05 between June 2019 and February 2020, visual comparison of parameter values and temporal trends shows little evidence of impact on River Clyde water chemistry. This initial surface water dataset shows little sign of mine water–surface water interaction, however further work is needed with mine water chemistry temporal monitoring data when those data are available, to further characterise the hydrological/hydrogeological system.
Rock Organic Carbon Characterisation and Dissolved Organic Matter Mobilisation Potential
Fluid-rock interactions are omnipresent processes in the subsurface, operating across micro to basin scales. Research into fluid-rock interactions has a long and diverse history (see Glassley et al., 2016 for overview). However, much of this research has focussed on inorganic inventories (e.g., Orem et al., 2014; Luek and Gonsior, 2017). Considerably less is known about organic matter (OM) pools that are mobilized in response to natural and engineered perturbations in the subsurface (e.g., Vieth et al., 2008; Wilke et al., 2015; Zhu et al., 2015), and whether rock-leached organic matter in pore water or induced waters leave a geochemical fingerprint that can be traced back to the source, preservation, level of thermal maturity, and potential reactivity of the OM in the original source rock. This information may be critical to better ascertain the impact of perturbed rock-water interactions due to either increased microbiological activity or organic-mineral interactions that could generate climate active gases such as methane (CH4) and carbon dioxide (CO2), or mobilise critical elements such as trace metals into the aquifer. These gases and organo-mineral complexes could potentially migrate and interact with the surface environment.
Here we present the first results from an investigation into water leachable organic matter (WLOM) from two mixed organic-rich seam transitions (Glasgow Upper coal-sandstone 1 from borehole GGA04 and Glasgow Main coal-mudstone transition from borehole GGA05) and an interlayered sandstone (sandstone 2 from GGA05) obtained as cuttings from three sequential horizons at site 2 of the Glasgow Observatory (location on Figure 3). The samples represent the main lithological end-members of Carboniferous strata in the subsurface, obtained from the during-drilling sampling opportunity.
Relative proportions of coal versus sandstone and mudstone cuttings in the transitional samples can influence the geochemical data. The coal-mudstone transition sample consists of 75% silty mudstone with siderite nodules and 25% coal, while the Glasgow Upper coal-sandstone 1 sample is 70% coal, 20% mudstone and 10% fine sandstone. The OM types of the organic-rich coal transitional samples (Supplementary Table S2) are thermally immature, evidenced by RockEval (RE). The %TOC of the upper coal-sandstone sample (50–51 mbgl) is 23.7% while the lower coal-mudstone transition sample (87–88 mbgl) is lower at 12.8%.
The coal fragments from the two horizons are strikingly different in their OM composition, as confirmed by petrography. Coal of the Glasgow Upper coal-sandstone 1 sample is from a terrestrial source with 77% vitrinite (humic coal) whereas the deeper coal-mudstone transition is primarily of algal source, confirmed by 80% of liptinite macerals (sapropel-type coal). The upper coal-sandstone 1 has higher Hydrogen Indices (HI, 195 mgHC/gTOC) compared to the much lower HI (93 mgHC/gTOC) in the coal-mudstone transition sample (Supplementary Table S2). The sandstone sample at 84–85 mbgl has elevated TOC (1.2%), very low HI (67 mgHC/gTOC) and a Tmax of 437°C. We attribute the elevated Tmax of the sandstone sample to the low S2 yield and therefore the uncertain definition of Tmax temperature. Despite notable difference in the organic composition in the coals itself the δ13C of all samples studied is rather homogenous, ranging from −22.8 to −23.5‰ (V-PDB). We initially interpret this observation as the result of the variable mixtures of coal with clastic lithologies.
To examine the potential for WLOM to provide diagnostic information on the OM content and composition we conducted a series of leachate experiments where rocks were mixed with deionised (18.2 MΩ cm−1), carbon free-water (1:12.5 wt/vol.) in Teflon tubes and placed in a shaking incubator, at a temperature of 50°C for 48 h. Resulting filtrates were analysed using liquid chromatography, organic carbon detection, organic nitrogen detection and UV absorbance detection (LC-OCD-OND-UVD; Huber et al., 2011; Zhu et al., 2015) to identify different dissolved OM (DOM) fractions (Figure 7) using size exclusion chromatography. Our results demonstrate the large potential of this technique to identify and characterise OC-rich lithologies (in this case study coal) from mixed transitional samples. We show that despite the coal-mudstone transition sample having a lower %TOC (Supplementary Table S2), it generated over three times more dissolved organic carbon (DOC; 152 ± 0.31 mg−1g rock) compared to the upper coal-sandstone 1 sample (44 ± 0.44 mg−1g rock; Figure 7). Kerogen microscopy identified a stark contrast between both coal transitions, with a distinct liptinitic signature for the coal-mudstone sample.
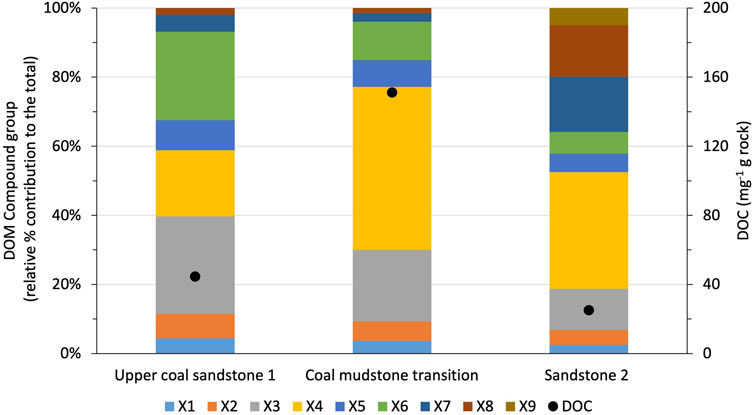
FIGURE 7. LC-OCD-OND-UVD water leachable organic matter compound groups identified from Site 2 of the Glasgow Observatory in different lithologies. DOM, dissolved organic matter; DOC, dissolved organic carbon; X1–X9 refer to identified DOM compound groups with increasing relative molecular weight. © Heriot-Watt University 2022.
The higher DOC in the coal-mudstone sample is primarily sourced from the SEC fraction X4 (low molecular weight acids; Zhu et al., 2015) likely reflecting higher release from sapropelic sources. Our LC-OCD-OND-UVD system distinguishes various low molecular weight group fractions (X5–X9) which have not been previously reported. These fractions are particularly enriched in the sandstone sample, where X7–X9 components contribute over 30% of the total DOC pool (Figure 7). Furthermore, the coal-mudstone transition sample has higher relative abundance of lower molecular weight neutrals (X4–X9) compared to upper coal-sandstone 1, which has higher X3 abundance. These initial results suggest distinct contrasts in leachable DOC from the different coal types, balanced against the clastic (mudstone or sandstone) background. The sandstone background has the lowest DOC potential (25 mg-1g rock) yet it displays a similar DOC composition to the coal-mudstone sample, suggesting a common OM source. Further work is planned to consolidate these first results, calibrate the experimental lab-based DOM end member profiles with flowback and groundwater samples collected during site construction and assess the potential of WLOM to contribute to diagnostic characterisation of subsurface rocks.
It is unknown whether and how WLOM may be accessed by microbial communities indigenous to the subsurface or introduced through fluid injection. As discussed below, microbial activity in the perturbed subsurface could lead to the generation of climate active gases as well as damage to infrastructure and operational issues such as hydrogen sulphide generation, corrosion, clogging and biofouling. The pioneering nature of integrating organic, inorganic, and microbial factors to assess the environmental impact of utilising subsurface resources for sustainable heat is required for comprehensive understanding of impacts from new low carbon development strategies. Therefore further work is necessary to assess the potential of WLOM from various lithologies to support microbial activity and establish how biodegradation affects WLOM profiles, and identify generic properties that offer diagnostic capability for improved operation of subsurface systems.
Bulk Gases and Stable Isotope Differentiation of Gas Sources
Hazardous ground gases, such as CH4 and CO2, can be found naturally in superficial deposits and coal bearing strata (Hall et al., 2005; CL:AIRE, 2021). Determining the presence, magnitude, and origin of mine gases, and how their geochemical fingerprints change through the shallow subsurface, is vital to developing an understanding of how to manage the risk posed by different ground gases in the sustainable development of geoenergy technologies (Simioni et al., 2021). Such potential risks of mine gas migration to local communities is exemplified by the events in the Scottish town of Gorebridge in 2013–2014, where mine gas ingress into residential houses resulted in a public health incident, and the subsequent demolition of a public housing estate comprising of 64 homes (Ramsay et al., 2017). Access to the Glasgow Observatory during the construction phase provided a unique opportunity to investigate the variability of the gas fingerprints with depth within the coal mine workings and unmined Carboniferous coal measures. Rock core samples were collected from the 199 m deep seismic monitoring borehole (GGC01) and drill cutting samples were obtained from both GGA05 and GGA08 (Figure 2). Samples were collected in gas tight isojars during the drilling programme after the methods outlined in Hendry et al. (2016, 2017), which were filled with distilled water leaving a small headspace and then stored for 2 months to allow degassing to occur. Geochemical gas analyses consisted of bulk concentration analysis using gas chromatography; followed by δ13CCH4, δ13CCO2, and δD stable isotopes, in order to determine potential gas origins.
The initial data obtained identifies the presence of both CH4 and CO2 in the gases exsolved from samples from all three boreholes (GGC01, GGA05, and GGA08). No correlation between gas concentration and depth was observed, with both CH4 and CO2 gas concentration values being highly variable and closely linked to stratigraphic horizon. For the unmined borehole (GGC01), both CH4 and CO2 gas were only detected at depths below 77 m. Samples with increased concentrations of CH4 gas appear to correlate to areas immediately surrounding unmined coal seams, with concentrations ranging from 6 to 88 mg/L (mean = 17 mg/L, Std. dev = 23 mg/L). Samples with the highest CO2 concentrations occur in samples where CH4 concentrations are lowest or absent, and range from 2 to 118 mg/L (mean = 33 mg/L, Std. dev = 37 mg/L). For mined boreholes GGA05 and GGA08, considerably fewer instances of CH4 gas were found to be present throughout the succession (9 out of 54 samples compared to 12 out of 15 samples from GGC01), with the majority of samples having levels below detection limits, which complements groundwater concentration data (Palumbo-Roe et al., 2021). In GGA08, CH4 was identified at four stratigraphic depths; all of which correspond to areas of coal seams or mine workings. In GGA05, CH4 was solely detected at 57–67 m depth in a cluster of samples, corresponding to the succession directly above the Glasgow Ell mine workings. CH4 concentrations for GGA05 and GGA08 boreholes ranged from 6 to 324 mg/L (mean = 53 mg/L, Std. dev = 102 mg/L), with the highest CH4 concentration occurring within a sample from the unmined Glasgow Ell Index coal seam in GGA05. These values are higher than in-situ groundwater CH4 concentrations recorded e.g., Glasgow Main (174–185 μg/L) and Glasgow Upper (117–145 μg/L) (Palumbo-Roe et al., 2021). CO2 gas is present throughout the succession of both GGA05 and GGA08, with concentrations ranging from 4 to 130 mg/L (mean = 31 mg/L, Std. dev = 30 mg/L), and correspond well with measured groundwater concentrations of 105–256 mg/L (Palumbo-Roe et al., 2021).
Samples from the unmined borehole GGC01 exhibit a narrow δ13CCH4 range of −73.4‰ to −64‰, with associated δDCH4 values between −277‰ and −240‰. This signature indicates a biogenic source of CH4, with carbonate reduction being the predominant generation pathway. The shallow mined boreholes GGA05 and GGA08 have a δ13CCH4 signature of −74.1‰ to −14.3‰ and δDCH4 of −259‰–17.3‰, with the majority of samples aligning with the biogenic signature exhibited by borehole GGC01. Samples enriched in 13C and 2H are found between depths of 63–79 m and may highlight CH4 oxidation in proximity to the Glasgow Ell coal mine workings (Figure 8). Initial data from this study indicate that associated CO2 gas has an enriched 13C signature relative to the CH4 present. δ13CCO2 values for GGC01 range between −12.7‰ and −6.1‰, with samples from GGA05 and GGA08 boreholes having a more depleted δ13CCO2 signature of −29‰ to −10‰. CO2 gas signatures become progressively depleted in 13C at shallower depths (above 90 m) as observed in boreholes GGA05 and GGA08 (Figure 8). The values recorded from the superficial deposits are the most depleted in 13C, and the unmined bedrock samples from GGC01 are the most enriched in 13C. This trend can be attributed to the increasing influence of shallow groundwaters that contain a mix of dissolved marine carbonate minerals (∼0‰) and soil gas CO2 (−26‰) as depth decreases.
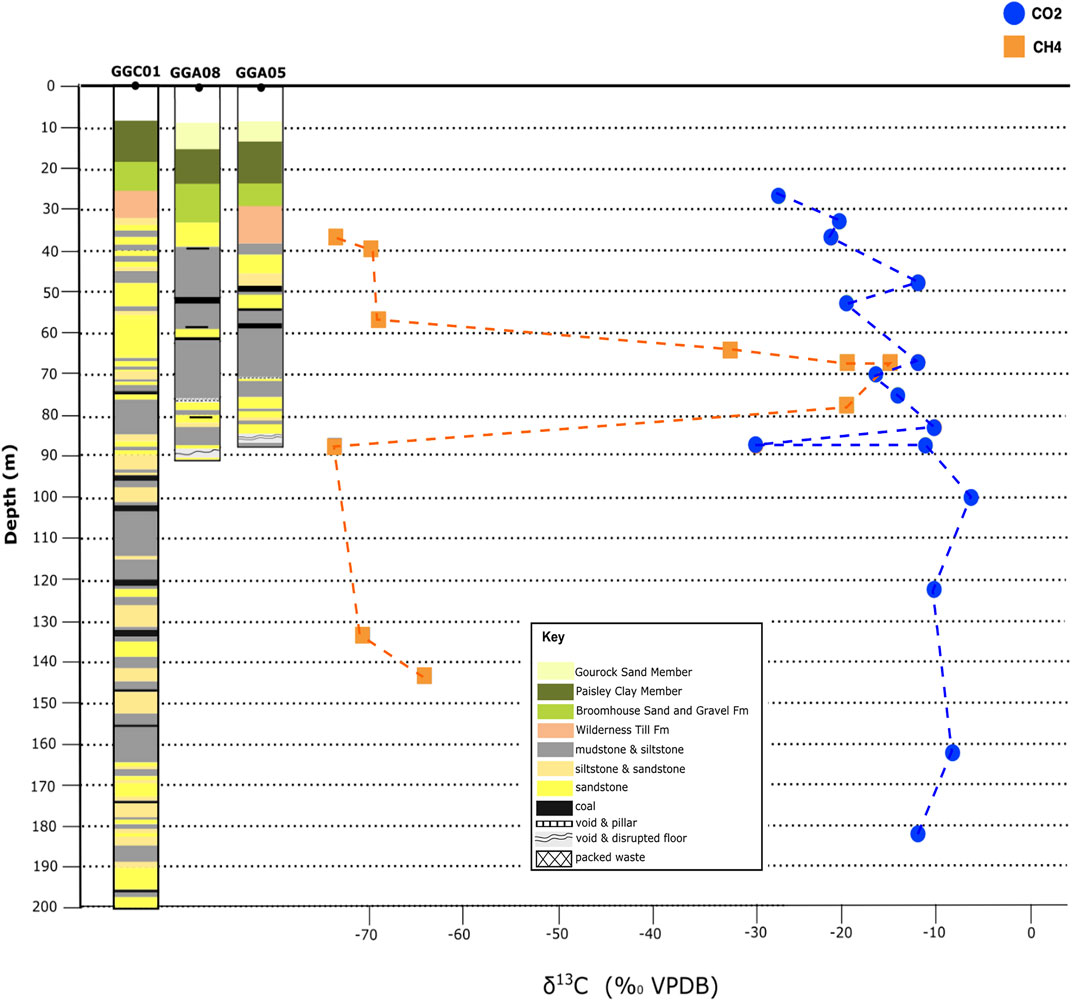
FIGURE 8. Isotopic depth plots of CH4 and CO2 δ13C values from GGC01, GGA05, and GGA08 boreholes; with the corresponding borehole stratigraphy. © Edinburgh University 2022.
In comparison to δ13CCO2, δ13CCH4 values exhibit no consistent variation with depth, with a notable zone of enriched δ13CCH4 occurring between 63 and 79 m (Figure 8). On-going work is aimed at fully resolving the gas generation pathways and enabling greater understanding of the mixing of gas sources within the Glasgow Observatory site, along with integration with microbiological analysis to identify the bacteria and conditions responsible for gas generation.
Oxygen, Hydrogen and Sulphur Isotopes
A variety of water samples (including borehole/return waters during drilling, from test pumping and surface waters), and subsamples of rock specimens from coring and drill cuttings were collected for the isotopic analyses of water molecules (δ2H and δ18O), sulphides (δ34S) and dissolved sulphate (δ34S) to determine the origins and behaviour of the waters, the source of sulphur (S) and to assess variability in isotopic composition across the Glasgow Observatory (Table 1, SUERC analyses). Details of the stable isotope analytical methods applied are given in Burnside et al. (2016a).
In line with previous studies across Europe and the data from pumping tests (Burnside et al., 2016a; Burnside et al., 2016b; Janson et al., 2016; Loredo et al., 2017), the δ18OH2O and δ2HH2O results during drilling and pump testing infer that mine water is dominated by recent meteoric recharge (Figure 9). The average value of surface waters measured during the drilling phase (δ18O of −7.7‰, and δ2H of −49‰) is directly coincident with the average of waters from the pump tests, δ18O of −7.6‰, and δ2H of −49‰. Pump test samples also fall within the local seasonal meteoric range (average δ18O of −7.6 ± 0.5‰, and δ2H of −49 ± 1‰; n = 15) and demonstrate no pump-related system perturbance with respect to δ18OH2O and δ2HH2O isotopes (Figure 9).
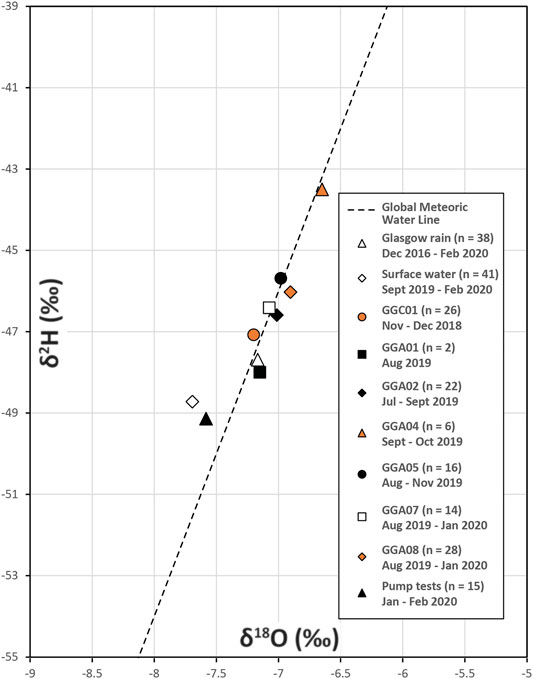
FIGURE 9. Average δ18OH2O and δ2HH2O results from during borehole drilling and pump tests across seven boreholes, surface waters and Glasgow rain waters as measured at SUERC. These latter data consist of 38 consecutive monthly samples collected from the roof of the Rankine Building, University of Glasgow [NS 57111 66792]). ©SUERC/University of Glasgow and Strathclyde University 2022.
Reconnaissance analyses of sulphides and sulphates have been measured for the first-drilled, cored GGC01 observation borehole (which encountered intact, non-worked coal seams). It was previously assumed that δ34SSO4 in mine waters reflects oxidation of parent sulphide minerals in coals (Banks et al., 2020), although recent work suggests more complex origins are likely, as revealed by highly variable and elevated δ34SSO4 values within mine water bodies (Burnside et al., 2016a; Burnside et al., 2016b; Janson et al., 2016). Establishing the cause(s) of δ34SSO4 variability within flooded mines would provide new insight into the hydrogeological behaviour of such systems (not revealed by more standard measures, such as δ18OH2O, δ2HH2O and hydrochemistry), possibly enabling better assessment of hydraulic connectivity and resource volume accessibility prior to and during pumped extraction. Sulphate in water samples of the non-mined borehole GGC01, taken from either the borehole prior to daily drilling activity or the return fluid settlement tank, returned δ34S values between −2.3 and 3.5‰ (n = 8;
Further S isotopic analyses will be required to establish sulphide baseline values for each of the worked seams; and to characterise the δ34SSO4 of pump-test waters to explore any lateral and/or depth variation in values, to provide a more comprehensive understanding of time zero in this mine water system. This will allow an assessment of compartmentalisation and its potential utility in assessing evolutionary trends during geoenergy extraction. Added value will arise from combining the S isotope data with the gas analyses and microbial analyses to assess the microbial impacts on the system.
Geomicrobiology
Microbes inhabit subsurface environments of temperatures below ∼120°C, and microbial metabolisms mediate the biogeochemical cycling of nearly all nutrients and trace metals either directly (e.g., via redox transformations) or indirectly (e.g., via influencing their adsorption/desorption on minerals or organic matter) (Banfield and Nealson, 1997; Chapelle, 2000; Ehrlich et al., 2002). The Glasgow mine water geothermal system comprises coal seams and mudstone horizons of the Coal Measures. Both coal and mudstone contain pyrite; microbial oxidation of pyrite generates acidity and ochre, and solubilises metals. Microbial respiration and fermentation of organic compounds leached from geological substrates forms CO2 and CH4 gases (Chapelle, 2000). Not only do these microbial products pose environmental hazards, they also may adversely impact on the operation of a mine water geothermal system.
A review of published literature found that changing groundwater flow conditions and temperatures may stimulate biofilm formation, which can clog pores and pipework, although most of our knowledge on biofilm formation comes from laboratory experiments, or studies of engineered environments such as drinking water systems (Clitherow, 2021). Studies of five natural surface waters all found that biofilm formation increased at low flow velocities (0.01–30 cm s−1); this trend was also observed in four studies of geothermal heat exchangers where biofilm formation was favoured in stagnant conditions created during plant downtime (Clitherow, 2021). Growth of microorganisms in thermal water systems has caused issues with reinjection to ground or corrosion of pumping equipment (Lerm et al., 2013; Wördemann et al., 2014; Osvald et al., 2017). Thermophilic bacteria have been found to contribute to bioclogging of above ground infrastructure at geothermal plants (Kim and Lee, 2019). Increased temperatures above 15 °C caused increased growth of heterotrophic bacteria biofilms in a geothermal heat pump (Smith and Liu, 2018). Studies of natural surface environments and geothermal energy systems all found increased carbon availability promotes biofilm formation, microbiological activity and diversity (Clitherow, 2021). There is a gap in our knowledge regarding how biofilms form in the subsurface; do these trends observed in the surficial and engineered systems apply to subsurface environments? Are they likely to have a significant effect on the operation of mine water geothermal systems? It is imperative that we understand the microbial community abundance, composition, and activity and how these factors might change as a result of fluctuating groundwater flow, temperature and geochemistry that are likely to occur during mine water system operation.
To investigate the microbial communities in baseline mine water groundwaters, 1 L samples were filtered at 0.22 and 0.1 µm and DNA was extracted (PowerWater kit, Qiagen). DNA yield was not detectable (Qbit assay). Following a polymerase chain reaction to amplify the marker gene for bacteria and archaea (16S rRNA amplicon), faint bands were visible using gel electrophoresis, indicating that these microorganisms were present in very small quantities in groundwater samples collected during drilling and pump testing. Indeed, after storage of the during drilling samples at 4°C for approximately 3 months, ochre flocs formed and Gram staining showed that microorganisms were present, suggesting that microbial growth occurred during storage conditions. Subsequent work will involve filtering larger volumes of fluid to yield greater quantities of DNA suitable for sequencing, as well as implementation of specialised DNA recovery protocols developed for low biomass-type samples. The results will be integrated with isotope geochemistry to identify microorganisms potentially responsible for producing CH4 measured at the site. Multivariate statistical analyses will be performed to test for correlation amongst phylogenetic and geochemical datasets; e.g., clustering of certain microbial DNA sequences may indicate greater abundance associated with certain geochemical conditions, organic matter source availability, groundwater flow rates or other environmental spatiotemporal variables.
Wider Environmental Baseline (Pre-, During, and Post-Drill)
Former coalfield areas can suffer from a range of mining legacy environmental impacts (subsidence, rising mine water and discharges, mine gas movement) and other types of geothermal technology interventions have been related to felt seismicity (e.g., Deichmann and Giardini, 2009). Monitoring infrastructure and surveys at the Glasgow Observatory has allowed the collection of relevant data required to assess these potential impacts.
Ground Gas
Ground (soil) gas measurement is an important tool for monitoring landfill and geoenergy activities, since sensitive measurements of key gases can be made directly, and visibly, within the biosphere in which we live. However, it has not previously been applied to shallow geothermal/mine water heat (Smith et al., 2021). Desorption of gas from coal and thus mine gas production and migration are significantly lowered within flooded mine systems (Appleton et al., 1995; Appleton, 2011; The Coal Authority et al., 2019), such as the flooded mine system at the Glasgow Observatory. Nevertheless, as part of a broad environmental monitoring effort, three ground gas baseline surveys were undertaken at the Glasgow Observatory to establish whether gases originating in the mine workings or from the complex overlying made ground, that might be cause for concern, could be measured in the near surface environment. Field measurements of CO2 and CH4 flux at the soil-atmosphere interface, and ground gas concentrations of CO2, CH4, H2, H2S, O2 and a proxy for N2 were taken across 20 m-spaced sampling grids, using established portable soil gas and gas flux methods i.e., hollow steel probes positioned to access gas at <70 cm bgl with end of pipe gas meters/sampling, and portable accumulation chamber flux [see Beaubien et al. (2013) for more details]. A small number of ground gas samples were also collected at <1 m below ground level for 13C/12C ratio determination in CO2 (Barkwith et al., 2020). Given the known complexity of the subsurface in this area, it is unlikely that the 20 m grid approach would detect all gas migration pathways connecting to the surface, especially if surface manifestation is highly discrete. Legacy boreholes are present but were not specifically targeted. However, survey grids were designed to encompass a mapped bedrock fault and there are no known shafts or adits to take account of. Tying survey data into wider temporal and spatial data is beyond the current scope, but is planned for further work.
Based on typical mine gas compositions of percentage-level concentrations of CH4 and CO2 with deficient O2 (Appleton et al., 1995), no evidence was found to suggest ground gas was unduly impacted by gas migrating from the workings. In detail, ground gas CH4 concentrations were comparable to atmospheric gas (<3 ppm by volume) and CH4 flux was typically below detection limits. CO2 flux (Figure 10A) was consistent with uncontaminated rural (Ward et al., 2019) and other UK sites surveyed by BGS, however isolated points with moderate ground gas CO2 concentrations (10–20% by volume) were inconsistent across surveys. Stoichiometric CO2:O2 relationships (Figures 10C–E) indicated a mixed natural origin of photosynthetic production, and microbial oxidation of CH4 to CO2 (Romanak et al., 2012); this is also supported by the limited number of available stable carbon isotope ratios, i.e., typically between –23.59 and –26.31 δ13CV- PDB, but with an isolated, unexplained enriched δ13C value of −16.76 at sample GG01-038 in May 2019 (data in Barkwith et al., 2020; interpretation based on Flude et al., 2017; Figure 10B). Isolated moderate values of H2 and H2S (<65 ppm and <38 ppm, respectively) were well below any potential explosion risk and since concentrations and compositions of CO2, CH4 and O2 in ground gas did not suggest a strong influence from mine gas, it is unlikely that H2 and H2S originated from mine gas either.
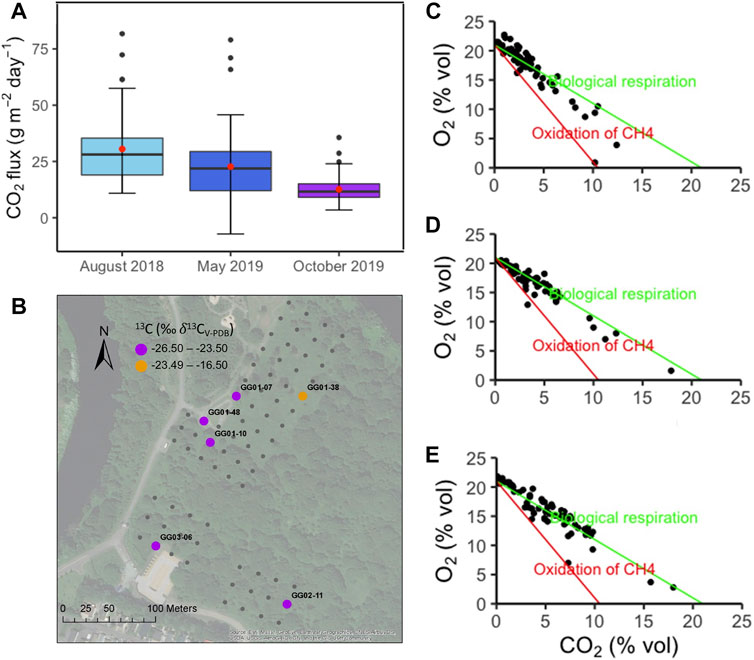
FIGURE 10. (A) Box and whisker plots of CO2 flux for three ground gas surveys at the Glasgow Observatory, all sites; (B) Spatial distribution of stable carbon isotopes in ground gas CO2 collected at <1 m below ground level; (C–E) Binary plots of ground gas O2 concentration as a function of CO2 for August 2018, May 2019 and October 2019 surveys respectively with process attribution after Romanak et al., 2012. Adapted from Smith et al. (2021 Figures 7, 11, 14) ©Crown Copyright (2021), with permission from Elsevier.
As a first attempt to apply established ground gas techniques to shallow geothermal/mine water heat in a complex post-industrial setting, valuable pre-operational data and a spatial context for future continuous (temporal) ground gas monitoring has been produced. The longer-term intention is to tie survey data into existing continuous soil gas monitoring and planned atmospheric monitoring to better account for diurnal and seasonal variability, and to interpret findings in the context of complimentary characterisation of the deeper subsurface (permeability, gas/fluid flow pathways) and monitoring of the surface environment, which would progress our ability to monitor for gases in a complex urban setting. The data reported in this paper provide a baseline against which future perturbations can be assessed. There is a clear need for geoenergy environmental monitoring techniques that take us beyond locating and quantifying, to enabling ground gas origins to be reliably attributed. This may be by studying isotopic characteristics from the near surface through a range of depths as detailed in borehole samples above, or the deployment of rapid multi-parameter screening at the surface.
Soil Chemistry
Soil geochemistry plays an essential role in environmental protection. Soil is sensitive to, and is a potential reservoir of, environmental pollution, particularly in urban areas and in relation to industrial activity (e.g., Fordyce et al., 2005; Broadway et al., 2010; Johnson et al., 2011; Alloway, 2013; Kim et al., 2019). The Glasgow Observatory soil chemistry dataset provides a necessary understanding of the soil quality and ground conditions to help satisfy regulatory requirements for the infrastructure installation, and to provide public reassurance. The premise is that development of a mine water geothermal facility should not instigate any material change in surface conditions. However, because a history of diverse human and industrial activities, including coal mining, has affected the subsurface at the Observatory sites, and due to the potential for changes in subsurface fluid flow to alter near-surface flow and soil saturation regimes and hence, soil chemistry, the soil geochemistry survey was essential to permit post-development monitoring of the near-surface environment and interactions with the subsurface. It is also intended to serve as an exemplar for similar schemes.
Topsoil sampling (0–20 cm depth) was carried out in February-March 2018 at the seven initially proposed Observatory borehole sites (boreholes were actually installed at five of these sites), and at two control sites with semi-natural soil in Glasgow Green and Tollcross Park (90 samples in total: Figure 2). The sampling and analytical methods followed those used in previous BGS soil surveys of Glasgow (Fordyce et al., 2012; Fordyce et al., 2017; Fordyce et al., 2019), and a related study of organic pollutants (Kim et al., 2019), to permit direct comparisons with existing BGS soil chemistry datasets. The methodology is summarised in Table 1 and results in Supplementary Table S3; after Fordyce F. M. et al. (2020).
The results show that topsoil from the Observatory sites generally contains higher inorganic and organic pollutant concentrations, is more alkaline, and contains greater concentrations of calcium, iron and magnesium (which commonly relate to building waste) than semi-natural topsoil collected from the two control sites in Glasgow Green and Tollcross Park. The maximum concentrations of the pollutants cadmium, TPH, the naphthalene and dibenzofuran PAH compounds, ∑7PCB [the sum of seven selected PCB congeners; see Kim et al. (2019)] and ∑tri-hepta PCB compounds reported at the Observatory sites exceed those in the city-wide BGS Glasgow topsoil datasets. These findings are deemed a consequence of historic land use at the Glasgow Observatory sites, all of which are situated on areas of extensive made ground that contains building rubble, domestic rubbish and/or colliery waste. Soil quality at the sites has demonstrably been polluted by the presence of these materials in the soil.
However, comparisons with current generic soil guideline values for assessing land contamination (see Supplementary Table S3; VROM, 2009; DEFRA, 2014; Nathanail et al., 2015) indicate that in general, land at the Observatory sites would not be classed as contaminated, with reference to guidelines for recreational open space. Although two soil samples collected outside the publicly accessible area at site 5 exceed the guideline value for lead (1300 mg/kg), further investigation is needed to determine if there is a source-pathway-receptor linkage. Hexavalent chromium [Cr(VI)] was measured because a history of disposal of chromite ore processing residue (COPR) elsewhere in the east of Glasgow has resulted in contamination of soil and surface/ground water with Cr(VI) (e.g., Broadway et al., 2010; Palumbo-Roe et al., 2017). There is no evidence of COPR waste in the topsoil samples collected from the Observatory sites; Cr(VI) concentrations in the soil were generally <10 mg/kg. Although 28 mg/kg was measured for one sample at site 6b, this is attributed to paint fragments present in the sample and this site was not developed for the Observatory.
Ground Motion
Before undertaking operations in the subsurface it is important to establish baseline for natural and anthropogenic ground motions (Novellino et al., 2021). This baseline acts as a benchmark for operational phases to provide reassurance that subsurface activities are not negatively impacting the stability of the surface (Jordan et al., 2019). In and around the Glasgow Observatory, the ground motion analysis is based on the interpretation of Interferometric Synthetic Aperture Radar (InSAR) satellite data acquired from the ERS satellite for 1995–2001, from ENVISAT satellite for 2002–2010 and from Sentinel-1 satellite for 2015–2017. To ensure the optimum resolution and density of InSAR measurement points, two InSAR processing algorithms were applied to the input satellite radar imagery:
(1) Intermittent Small Baseline and Subset (ISBAS), a patent pending algorithm developed by ©Geomatic Ventures Limited and whose IP belongs to the University of Nottingham—United Kingdom (Sowter et al., 2013; Bateson et al., 2015).
(2) SqueeSAR™, the proprietary multi-interferogram technique patented by TRE ALTAMIRA (Ferretti et al., 2011).
For a comparison of these methods, as applied to Glasgow, see Sadeghi et al. (2021).
The InSAR baseline analysis has revealed notable small areas of ground motion over the wider Glasgow area. These appear to relate to both natural (volume change of peat deposits, compressible ground) and anthropogenic (settling of made ground and landfill) factors (Figure 11A). No evidence was found for ground motions relating to the coal mining history of this area (Bateson and Novellino, 2019). For example, we do not see the regional patterns of uplift and subsidence that has been observed in InSAR results for other UK former coal mining areas on cessation of pumping and with mine water rebound (e.g., Gee et al., 2017; Gee et al., 2020). This is likely to be due to the age of mining, which stopped in the late 19th and early 20th century in urban parts of Glasgow, therefore such motions will have occurred prior to the availability of the SAR data. At the local scale, the Observatory sites show overall stability, small areas west of Cuningar Loop show minor amounts of subsidence, with rates of ∼5 mm/yr for 2015–2017 (Bateson and Novellino, 2019; Figure 11B). This motion is interpreted to relate to settling of relatively thick superficial and anthropogenic deposits, which were built upon for the development associated with the 2014 Commonwealth Games.
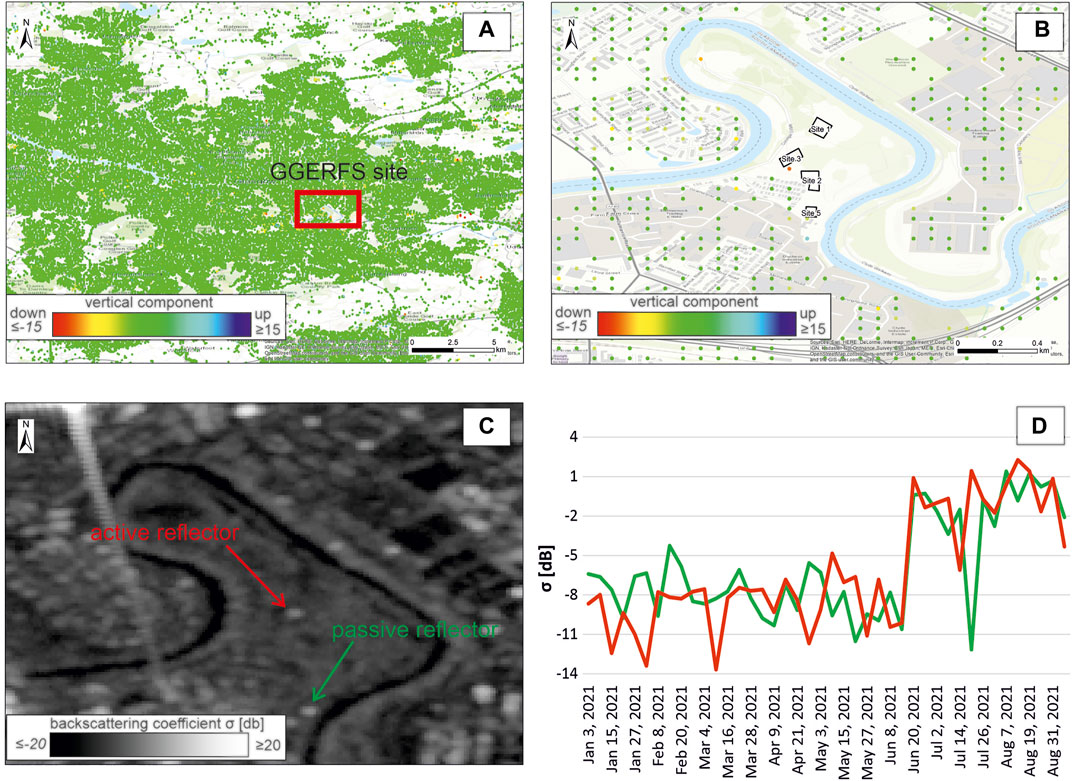
FIGURE 11. TRE ALTAMIRA SqueeSAR™ Sentinel 1 average vertical motions for (2015–2017) over Glasgow area (A) and Cuningar Loop portion of the Glasgow Observatory (B). Median of the backscattered signal (σ0) for the ascending Sentinel-1 acquisitions in July 2021 highlighting the impact of the active and passive reflectors installed (C). Time series for the backscattering coefficient (σ0) over the passive and active reflectors along the ascending Sentinel-1 acquisitions (D). Contains processed Copernicus Sentinel-1 data [2015–2017, 2021].
This characterisation of the ground motion in the Glasgow area for the last 3 decades forms the baseline to monitor any potential effects of the planned geothermal research activities. Further InSAR investigations with the Sentinel-1 constellation are planned to be conducted once the abstraction and re-injection of mine water starts. Active and passive radar corner reflectors were installed in the Cuningar Loop in June 2021 at Site 1 and Site 5. These will facilitate the calibration of the SAR imagery and increase the radar backscattered signal which will guarantee more accurate and precise InSAR measurements directly above subsurface operations (Figures 11C,D).
Seismic Monitoring
Seismic monitoring is in place to detect and locate any felt seismicity near to the Glasgow Observatory. Although planned activities are not expected to cause seismicity, it was considered desirable to have monitoring in place, if only to reassure the public that coincidental vibrations are not earthquakes induced by the research activities. Seismic monitoring in cities is well known to be problematic, due to the level of environmental noise being typically extremely high. Using boreholes greatly increases the signal-to-noise ratio in such cases, but such boreholes are expensive and require surface infrastructure. The solution utilised here was to install 5 seismometers in a single 200 m borehole approximately 2 km from the Observatory site at Cuningar Loop. Data from this array is openly available to view and download via ukgeos.ac.uk. It was incorporated into the work of Lecocq et al., 2020 that characterised global quietening of seismic noise during the first Covid pandemic lockdown (March-May 2020).
Functioning as a single station, the Observatory array, in combination with other stations of the UK National Seismic Network, ensures that any earthquake near the site will be detected down to a magnitude slightly less than 2. However, smaller earthquakes than this can be felt (Thouvenot and Thouvenot and Bouchon, 2008), and a novel detection algorithm has been developed and implemented to utilise the five sensors in the vertical array (Luckett, 2021). This works using the principle that seismic waves from an earthquake will arrive at the bottom seismometer first. A magnitude 0.5 ML earthquake occurred only 30 km from the Observatory soon after installation, allowing the new algorithm to be tested. Figure 12 shows the arrivals at the five seismometers with the bottom seismometer at the top. Once detected, the signal from a small earthquake can be looked for on the nearest seismometers of the National Network and a location determination carried out. If there is nothing on any other seismometer then a single station location can be completed using only the borehole data (e.g., Frohlich and Pulliam, 1999).
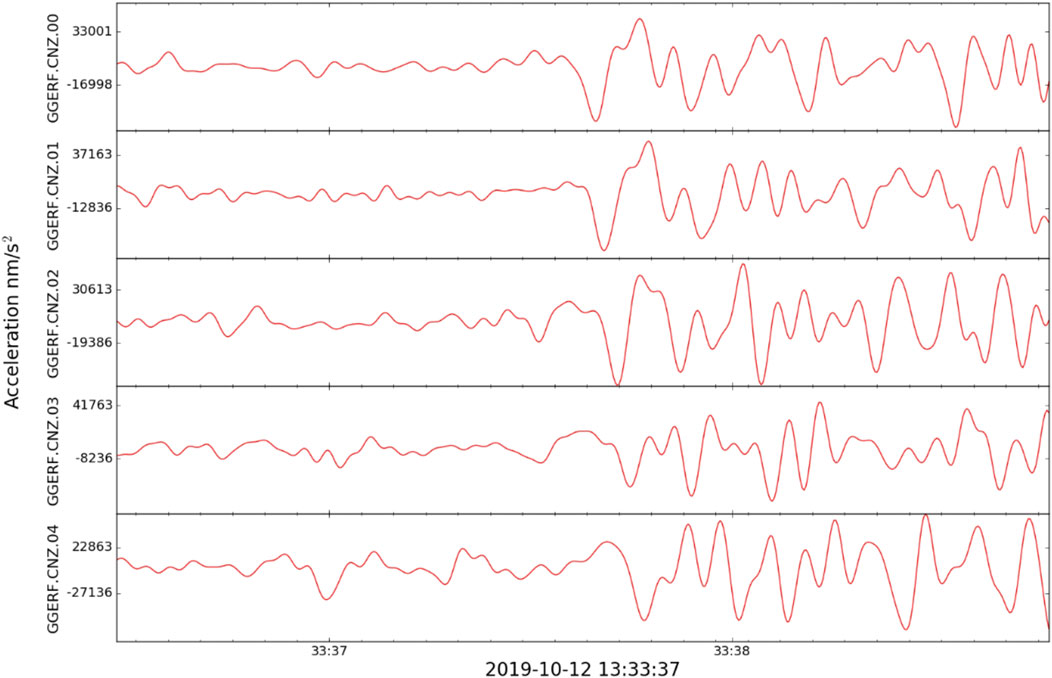
FIGURE 12. Acceleration time series on the vertical components of the 5 seismometers in borehole GGC01 of the Glasgow Observatory for the Barnacarry earthquake on 12th October 2019. Filtered between 1.25 and 45 Hz. The bottom seismometer is at the top of the plot.
Synthesis and Discussion
Conceptual Hydrogeological Model
The multidisciplinary approach adopted to data collection and analysis from drilling, testing and sampling have contributed to the development of a conceptual hydrogeological model of the top 90 m of the subsurface at the Glasgow Observatory site. This understanding is critical for geothermal activities that involve abstraction and re-injection of groundwater. The groundwater system at Cuningar Loop, Glasgow has been highly modified, with the lithologically-variable bedrock being affected by faulting and glaciation before modifications in the last few centuries due to mining and urbanisation (see hydrogeology section above). The site is also located next to the River Clyde, one of Scotland’s largest rivers (see surface water section above).
Groundwater flow in this shallow system is dominated by the abandoned, flooded mine workings (Figure 13). Both the aquifer testing and the water chemistry analysis indicate that in its relatively undisturbed state, flow is predominately laterally through individual mine workings; likely in a combination of flow through voids and waste in the workings, and fractures in the surrounding rock. There may be some interaction with intergranular storage in adjacent sandstones. Individual mine water boreholes can yield high flows of >20 L/s with temperatures in the mine workings of 11.5–12.5°C. There is evidence that individual mine workings are connected–possibly through mine shafts, stone roadways or faults, and that pumping in one mine working effects some changes in another. The sandstone immediately above the Glasgow Upper mine workings is strongly connected to the mine workings with both the aquifer properties and strength of connectivity interpreted to be modified by fractures induced by mining activity (Figure 13). The 5-h pumping tests conducted thus far do not provide evidence for groundwater in the shallow superficial deposits, above the Paisley Clay Member, being directly connected to the bedrock aquifers, though this may be a consequence of the pumping rate and test duration. However, the upward flow of groundwater as inferred by the gradient in piezometric heads, and the high levels of mineralisation in groundwater of the superficial deposits are consistent with discharge to the superficial deposits, possibly indirectly through vertical mine shafts and associated drainage conduits. Highly mineralised groundwater can also occur in Glasgow due to the presence of made ground including mine waste infill (Ó Dochartaigh et al., 2019), so further research is required to confidently attribute the observed elevated levels of groundwater mineralisation in shallow superficial deposits to mine water discharge.
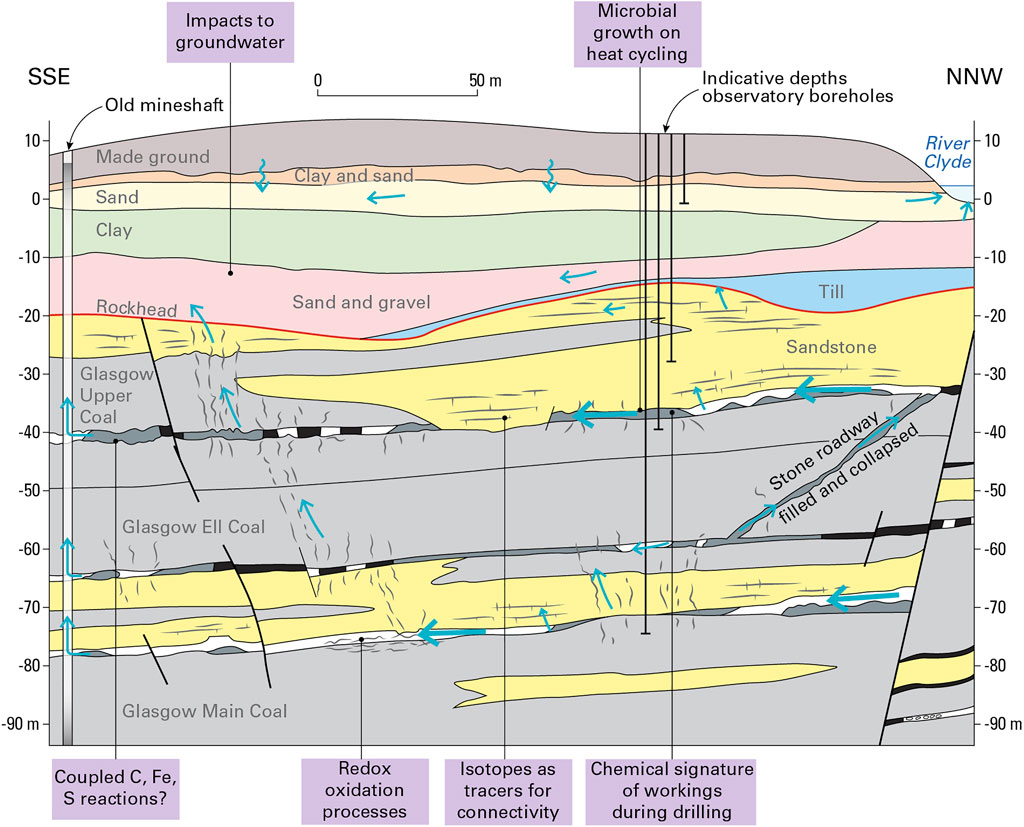
FIGURE 13. Summary conceptual hydrogeological model of mined Coal Measures in the vicinity of the Glasgow Observatory with representative features shown. Blue arrows indicate groundwater flow. Mine workings are water filled, grey within mine workings is mining waste, black is intact coal. In purple annotation, examples of techniques and processes identified during time-zero characterisation that may be impacted by heat and flow cycling due to geothermal operations.
The stable isotopes and residence time indicators suggest that the mean residence time of the groundwater in the system is 50–70 years (Palumbo-Roe et al., 2021), and that locally the system is not strongly connected with the atmosphere. Groundwater recharge is likely to be occurring within a few kilometres of the site, with groundwater storage in the bedrock and mine workings, and the high transmissivity of voids, backfilled waste and partially collapsed mine workings providing rapid transport. The residence time indicators suggest that the youngest groundwater may be found in the deeper mine workings, which would be consistent with this being a discharge area and general upward flow.
The deeper Glasgow Main mine working has higher transmissivity than the shallower Glasgow Upper mine working. This likely reflects a larger proportion of open void space remaining in the lower workings, possibly relating to the type and age of mining, lesser amount of stowage used and the strong sandstone roof of the mine working. Analysis of pumping tests and groundwater chemistry results from different boreholes within the individual mine workings gave similar results, despite being several hundred metres apart and (for the Glasgow Upper) encountering three different materials: coal pillar, mine waste and a void. This illustrates how the effects of localised heterogeneity in the hydraulic properties of the mine workings are averaged out as the cone of depression extends and integrates the variable properties of the mines. The local hydraulic conductivity significantly impacts drawdown in individual boreholes in the Glasgow Upper mine working.
The initial conceptual model (Figure 13) indicates that this site is typical of many shallow mine workings in Scotland. Flow in the mine workings is considerable, recharge relatively local and groundwater chemistry moderately mineralised with elevated iron, manganese and alkalinity. Groundwater temperature is slightly elevated above Scottish averages (MacDonald et al., 2017), attributed to the local geothermal gradient which has been impacted by the mining and also the industry and urbanisation (Watson and Westaway, 2020). Flow rates from initial test pumping and borehole spacings are representative of those of a small scale mine water heat scheme [when compared to larger schemes at Heerlen, Netherlands (Verhoeven et al., 2014) or Gateshead, United Kingdom (Steven, 2021)]. As such, the site can be regarded as representative to undertake further geoenergy-related research which aims to examine typical shallow coal mined environments in Carboniferous strata of the United Kingdom.
Initial Integration
The motivation for the site operator (BGS) to establish a baseline of ongoing environmental change prior to geothermal research commencing included environmental protection, public concerns and characterisation for future research users (Monaghan et al., 2018). Soil chemistry data highlights the variable quality of the made (artificial) ground; ground gas surveys indicate dominance of photosynthetic and microbial oxidation processes measured in the top few metres (as opposed to bedrock gas signatures that were measured as peaking around coals and mine workings) and surface water chemistry is dominated by seasonal and rainfall variations. How the relatively recent groundwater dominated by flow in the mine workings interacts with shallower groundwater and surface water, superficial deposits and soils remains to be further assessed once temporal groundwater data is available.
Multidisciplinary, explorative studies from the “during drilling” sampling programme have brought together a geochemical and microbiological toolbox that sheds light on coupled reactions in this complex subsurface system. For example, CH4 and CO2 isotopic data on exsolved bedrock gases is indicative of both oxidation processes and biogenic processes in the subsurface, as well as signatures that are characteristic of particular horizons (Figures 8, 13). Sulphur isotopic data contrasts between unmined and mined boreholes and accords with signatures observed in other mined systems. However, the causal processes and their linkage, such as biogenic and chemical redox reactions and groundwater sources remains to be fully investigated. In addition, during drilling samples have recorded the mobilisation of organic carbon, which may further impact on microbial growth and may affect subsurface C, Fe and S biogeochemical cycling (Figure 13).
Moving on From the Time-Zero Baseline to Applications for Shallow Geothermal Energy
The integration of geochemical, hydrogeological and microbiological characterisation and monitoring techniques at-scale in a real, complex urban system and during drilling and testing of boreholes for geothermal energy, has provided insights into process understanding of coupled rock-water-gas systems. Moving forward to development of other geothermal sites or geothermal operations, the time zero data and techniques could contribute in three main areas towards reducing costs and risks for the exploration, development and operations of mine water heat schemes.
Planning and Construction Risks: Accessing the Heat Resource and Resource Sustainability
Borehole drilling costs and risk on the accessible size, interconnectivity and sustainability of the water and heat resource are challenges for mine water heat projects (NELEP, 2021; Townsend et al., 2021; Walls et al., 2021). At a technical level, identifying and correlating mine workings and understanding how the mine water reservoir is connected can be complex to unravel; yet critical to the size and sustainability of the resource. Hydrogeological pumping tests with monitoring of observation boreholes are essential. In addition, we have highlighted a suite of geochemical techniques that have potential as a toolbox of “fingerprinting” techniques during drilling and exploration. These could inform the resource connectivity at relatively low cost and act as “tracers” both constraining the heat resource and beginning the characterisation of natural and induced groundwater flow. Three examples of novel monitoring approaches include:
1) Depth-dependent isotopic gas fingerprints in a mined succession (Figure 8) and different, distinctive ground gas isotopic compositions. For example, whether specific coal seams and/or mine workings have distinctive gas signatures that can be elucidated within the complexity of sources, pathways and receptors in a flooded, faulted and mined subsurface. Once pumping, heat abstraction and re-injection commence these distinctive signatures may be used to evaluate any induced changes in the subsurface.
2) Isotopic O, H and C signatures provide information on the meteoric water recharge into the groundwater. However, δ34SSO4 behaves differently and provides new insight into the hydrogeological behaviour, redox state, ultimately enabling better assessment of hydraulic connectivity (Figure 13) and resource volume accessibility prior to pumped extraction.
3) DOM profiles of water leachates have potential applications for during-drilling diagnostic characterisation of circulating drilling waters for correlation and characterisation of key horizon and reservoir intervals (Figure 7).
Geomicrobiological characterisation may also prove valuable for “fingerprinting” mine workings and surrounding aquifers, once methodological challenges for low biomass samples have been overcome.
Minimising Operational Costs and Risks: Understanding Biogeochemical Systems and Induced Changes
Economic margins for mine water heat schemes can be challenging and there is not an evolved supply chain for maintenance (Optimat, 2019; NELEP, 2021; Walls et al., 2021), so minimising operational costs and risks are of particular importance. Scaling and clogging of mine water systems and corrosion of geothermal infrastructure are well documented (Banks, 2009; Walls et al., 2021). There are a set of research challenges to better understand, mitigate and treat the impacts of hydrogeological, thermal perturbations, and induced chemical and microbiological changes in a mined subsurface on operational infrastructure. The time zero datasets presented in this paper give the baseline from which to monitor induced changes such as coupling of C, Fe and S reactions, evolving redox states and microbial mediation of biogeochemical reactions. For example, do changes in heat and flow associated with geothermal operations enhance organic leachates, geomicrobial activity and biofilms and/or gas production (Figure 13), and does that in turn cause oxidation of pyrite? It may be possible to develop biogeochemical and environmental quality indicator parameters to warn if adverse processes take hold, and to design mitigation measures.
Environmental Impact: Monitoring, Prediction, Mitigation
The vulnerability of the surface and shallow subsurface water chemistry regime to flow and heat perturbations associated with mine geothermal energy use is not currently well understood. For example, changes in flow paths, chemical changes and pollutant migration may impact water quality. In highly populated urban areas, social approval is a key challenge and, together with regulation, can be underpinned by robust environmental and geoscientific data.
The current work describes an exceptionally characterised real-world mined, urban system, poised to measure induced changes from geothermal activities. The high spatial and temporal resolution baseline datasets presented begin to reveal the complexity of the groundwater and surface water chemistry, the subsurface oxidation-reduction and biological processes that influence gas and isotopic signatures in rocks and soils. Further analysis of this time zero data will inform key monitoring parameters, predicted (and subsequently measured) effects under repeated heat and flow cycling of geothermal operations. Integration of water, gas, rock datasets will inform risk mitigation at vulnerable locations and mitigate against a disproportionately cautious approach at other locations. For example, monitoring of water chemistry for sulphates and sulphides, dissolved organic carbon and a range of C, H, O, S isotopes as well as standard inorganic elements and ions appears to be particularly important. Should this prove to be the case, new analytical monitoring technologies may follow to improve practicality and affordability.
Conclusion
Diverse time zero environmental monitoring and geochemistry datasets collected before, during and after borehole drilling and testing at a mine water geothermal research observatory in Glasgow, UK are providing unprecedented insights into coupled rock-water-gas-microbiological interactions, and their significance for mine water thermal resource development.
Initial assessment of rock organic carbon mobility, water and gas isotopic characterisation and geomicrobiology studies highlight distinctive isotopic, organic carbon and gas chemical signatures varying with depth in the subsurface and between boreholes. Biogeochemical interactions of S, C and Fe appear particularly important, possibly with microbially-mediated mineral oxidation/reduction reactions.
Time zero environmental characterisation has greatly increased the evidence base within an urban and formerly industrialised setting at the UK Geoenergy Observatory in Glasgow, before geothermal research commences. Groundwater and aquifer properties appear to be typical of mined Carboniferous strata and of urban superficial deposits; complex subsurface flow types and pathways are envisaged. While strong seasonal trends in surface water chemistry are apparent, there are no indications of changes associated with the Observatory construction, though further work is needed to investigate the interaction between deeper groundwater with upwards piezometric head and surface waters. Topsoil from Observatory sites generally contains higher inorganic and organic pollutant concentrations than city-wide datasets but principally remains below levels classed as contaminated for recreational open space. Ground gas does not appear to be impacted by gas migration from the mine workings, and provides an essential baseline to identify if such gas migration occurs in the future. InSAR ground motion data indicates stability in the vicinity of the Observatory boreholes but gradual subsidence nearby, and seismic monitoring has enhanced the resolution of the national network in the urban area.
Open data and scientific understanding form the core of social approval, with public concerns likely to be heightened in urban settings close to homes and businesses. Geoscientists increasingly are aware of their role in the whole energy chain and this work forms an unusual example of integrative multidisciplinary working. Together we have presented potential for a novel toolbox of biogeochemical and geoscientific monitoring techniques that could be transferable to key research challenges in earth system science for shallow geothermal technologies, namely 1) planning and construction resource risks where chemical and isotopic tracers can be used for characterisation and connectivity 2) operational maintenance and resource sustainability with better understanding of processes induced by heat and flow cycling such as potential for biofilms 3) regulation and monitoring of environmental impacts at heterogeneous sites. By increasing the evidence base, developing new, potentially lower-cost techniques, and informing the design of fit-for-purpose monitoring approaches, this could form part of the cost and risk reduction necessary for mine water heat and heat storage to form an important component in decarbonising heating of our buildings towards Net Zero greenhouse gas emissions.
Data Availability Statement
The datasets presented in this study can be found in online repositories. The names of the repository/repositories and accession number(s) can be found in the article/Supplementary Material.
Author Contributions
AMG: conceptualisation, investigation, project administration, writing, and visualisation; AB, NB, JdR, SG, DM, AMD, JM, LN, BP-R, RP, and HT-C: conceptualisation, investigation, writing, and visualisation; LB, RC, ED, PE, RL, and AN: investigation, writing, and visualisation; MJ, GJ, DS, MS, VS, CV, TW, and DW investigation and reviewing writing.
Funding
BGS author contributions using Natural Environment Research Council (NERC) national capability funding, utilising the UK Geoenergy Observatories infrastructure that was capital-funded by UK Government, through UK Research and Innovation and NERC. NB, AB, and DW: stable isotope work was funded by NERC NEIF grant number 2301.0920 and supported by analyses at the ICSF at SUERC (NERC Facility contract F14/G6/11/01). LN and RC received funding via a NERC project training opportunity (NU-000072). MJ and TW acknowledge support from the Petroleum Technology Development Fund (PTDF; Reference: PTDF/ED/PHD/JSM/1365/18). RP acknowledges financial support to the European Research Council BOOGIE project under the European Union’s Horizon 2020 research and innovation program (grant number 949495). GJ acknowledges support from the Faculty of Engineering at the University of Strathclyde.
Conflict of Interest
Authors AAM, LB, ED, PE, RL, DJM, AM, AN, BP-R, MS, VS, HT-C, and CV were employed by the British Geological Survey, a research centre of the Natural Environment Research Council.
The remaining authors declare that the research was conducted in the absence of any commercial or financial relationships that could be construed as a potential conflict of interest.
Publisher’s Note
All claims expressed in this article are solely those of the authors and do not necessarily represent those of their affiliated organizations, or those of the publisher, the editors, and the reviewers. Any product that may be evaluated in this article, or claim that may be made by its manufacturer, is not guaranteed or endorsed by the publisher.
Acknowledgments
Many former and current BGS colleagues have contributed to the delivery of the UK Geoenergy Observatory in Glasgow and the datasets described here including G. Baxter, R. Dearden, J. Burkin, C. Chapman, C. Buchanan, T. Galley, J. Midgley, B. McLean, B. Ó Dochartaigh, F. Fordyce, C. Watson, P. Tvaranavicius, B. Lister, S. Burke, C. Abesser, J. Elsome, K. Walker-Verkuil and K. Shorter. M. Schiltz of the University of Strathclyde is thanked for his assistance in sample collection and analysis. Z. Shipton and M. Knapp of the University of Strathclyde are thanked for their early input and discussions regarding the science and for facilitating sample storage and analysis. The Lyell Centre gratefully acknowledge the technical assistance provided by J. Bischoff. CA and AC are thanked for reviews of this paper, plus JR as Editor. This paper is published with the permission of the Executive Director, British Geological Survey (UKRI).
Supplementary Material
The Supplementary Material for this article can be found online at: https://www.escubed.org/articles/10.3389/esss.2022.10054/full#supplementary-material
Supplementary Table S1 | Overview of samples and analyses per borehole and per research group/analysis type. Figure 4 provides a simplified overview of this timeline.
Supplementary Table S2 | Rock characterisation parameters from Rock-Eval 6 and petrography analysis.
Supplementary Table S3 | Summary of Glasgow Observatory and BGS Glasgow soil survey results for selected major elements, inorganic and organic contaminants. See Fordyce et.al. (2017, 2019, 2020a, 2020b), Kim et. al. (2019). The generic guideline values for selected pollutants apply to recreational open space, and are taken, where these exist, from current UK human health risk assessment land contamination guidance published as: ‘Category 4 Soil Screening Levels’ for As, Cd, Pb, Cr(VI) and benzoapyrene (DEFRA 2014); ‘Suitable for use screening levels’ for Cr, Cu, and Ni (Nathanail et. al. 2015), and; a ‘trigger value’ for Σ7PCB (VROM 2009).
References
Abesser, C. (2020). Deep Impact: Unlocking the Potential of Geothermal Energy for Affordable, Low-Carbon Heating in the UK. Nottingham, UK: British Geological Survey. Available at: https://www.bgs.ac.uk/download/science-briefing-note-deep-geothermal/.
Abesser, C., and Walker, A. (2022). Geothermal Energy. POST Brief 46. Available at: https://researchbriefings.files.parliament.uk/documents/POST-PB-0046/POST-PB-0046.pdf.
Adams, C., Monaghan, A., and Gluyas, J. (2019). Mining for Heat. Geosci. 29 (4), 10–15. doi:10.1144/geosci2019-021
Alloway, B. E. (2013). “Sources of Heavy Metals and Metalloids in Soils,” in Heavy Metals in Soils. Environmental Pollution Series. Editor B. E. Alloway ((Netherlands: Springer), 11–50. doi:10.1007/978-94-007-4470-7_2
Appleton, J. D., Hooker, P. J., and Smith, N. J. P. (1995). Methane, Carbon Dioxide and Oil Seeps from Natural Sources and Mining Areas: Characteristics, Extent and Relevance to Planning and Development in Great Britain. Technical Report, WP/95/1. Keyworth, Nottingham: British Geological Survey.
Appleton, J. D. (2011). User Guide for the BGS Methane and Carbon Dioxide from Natural Sources and Coal Mining Dataset for Great Britain. Open Report OR/11/054. Nottingham, UK: British Geological Survey. Available at: http://pubs.bgs.ac.uk/publications.html?pubID=OR11054.
Arkley, S., and Callaghan, E. (2021). Model Metadata Report for the Glasgow Observatory Post-drill Superficial Deposits Model. Open Report, OR/21/034. Nottingham, UK: British Geological Survey, 52. Available at: http://nora.nerc.ac.uk/id/eprint/531155/.
Banfield, J. F., and Nealson, K. H. (Editors) (1997). Geomicrobiology: Interactions between Microbes and Minerals (Berlin, Germany: Walter de Gruyter GmbH & Co KG), 8.
Bailey, M. T., Gandy, C. J., Watson, I. A., Wyatt, L. M., and Jarvis, A. P. (2016). Heat Recovery Potential of Mine Water Treatment Systems in Great Britain. Int. J. Coal Geol. 164, 77–84. doi:10.1016/j.coal.2016.03.007
Banks, D., Athresh, A., Al-Habaibeh, A., and Burnside, N. (2017). Water from Abandoned Mines as a Heat Source: Practical Experiences of Open- and Closed-Loop Strategies, United Kingdom. Sustain. Water Resour. Manag. 5, 29–50. doi:10.1007/s40899-017-0094-7
Banks, D., Boyce, A. J., Burnside, N. M., Janson, E., and Roqueñi Gutierrez, N. (2020). On the Common Occurrence of Sulphate with Elevated δ34S in European Mine Waters: Sulphides, Evaporites or Seawater? Int. J. Coal Geol. 232, 18. doi:10.1016/j.coal.2020.103619
Barkwith, A., Lister, B., Taylor-Curran, H., Hannis, S., Shorter, K., Walker-Verkuil, K., et al. (2020). Data Release Notes : UK Geoenergy Observatories Glasgow Geothermal Energy Research Field Site (GGERFS) Ground Gas, 2018 and 2019 Surveys. Open Report, OR/20/037. Nottingham, UK: British Geological Survey, 16. Available at: http://nora.nerc.ac.uk/id/eprint/528737/.
Bateson, L., Cigna, F., Boon, D., and Sowter, A. (2015). The Application of the Intermittent SBAS (ISBAS) InSAR Method to the South Wales Coalfield, UK. Int. J. Appl. Earth Observation Geoinformation 34, 249–257. doi:10.1016/j.jag.2014.08.018
Bateson, L., and Novellino, A. (2019). Glasgow Geothermal Energy Research Field Site : Ground Motion Survey Report. Report No. OR/18/054. Nottingham, UK: British Geological Survey, 35. Available at: http://nora.nerc.ac.uk/id/eprint/524555/.
Beaubien, S. E., Jones, D. G., Gal, F., Barkwith, A. K. A. P., Braibant, G., Baubron, J. C., et al. (2013). Monitoring of Near-Surface Gas Geochemistry at the Weyburn, Canada, CO2-EOR Site, 2001 –2011. Int. J. Greenh. Gas Control 16, 236–S262. S2013. doi:10.1016/j.ijggc.2013.01.013
Birkel, C., Helliwell, R., Thornton, B., Gibbs, S., Cooper, P., Soulsby, C., et al. (2018). Characterization of Surface Water Isotope Spatial Patterns of Scotland. J. Geochem. Explor. 194, 71–80. doi:10.1016/j.gexplo.2018.07.011
Bracke, R., and Bussmann, G. (2015). “Heat-storage in Deep Hard Coal Mining Infrastructures,” in Proceedings World Geothermal Congress 2015, Melbourne, Australia, 19th–25th April 2015.
Broadway, A., Cave, M. R., Wragg, J., Fordyce, F. M., Bewley, R. J. F., Graham, M. C., et al. (2010). Determination of the Bioaccessibility of Chromium in Glasgow Soil and the Implications for Human Health Risk Assessment. Sci. Total Environ. 409, 267–277. doi:10.1016/j.scitotenv.2010.09.007
Browne, M. A. E., and McMillan, A. A. (1989). Quaternary Geology of the Clyde Valley. British Geological Survey Research Report SA/89/1 https://pubs.bgs.ac.uk/publications.html?pubID=B04083.
Bullock, L. A., Parnell, J., Perez, M., Boyce, A., Feldmann, J., and Armstrong, J. G. T. (2018). Multi-stage Pyrite Genesis and Epigenetic Selenium Enrichment of Greenburn Coals (East Ayrshire). Scott. J. Geol. 54, 37–49. doi:10.1144/sjg2017-010
Burnside, N. M., Banks, D., Boyce, A. J., and Athresh, A. (2016b). Hydrochemistry and Stable Isotopes as Tools for Understanding the Sustainability of Minewater Geothermal Energy Production from a ‘standing Column' Heat Pump System: Markham Colliery, Bolsover, Derbyshire, UK. Int. J. Coal Geol. 165, 223–230. doi:10.1016/j.coal.2016.08.021
Burnside, N. M., Banks, D., and Boyce, A. J. (2016a). Sustainability of Thermal Energy Production at the Flooded Mine Workings of the Former Caphouse Colliery, Yorkshire, United Kingdom. Int. J. Coal Geol. 164, 85–91. doi:10.1016/j.coal.2016.03.006
Busby, J., and Mansor, N. (2021). IEA Geothermal, UK 2020 Country Report. https://iea-gia.org/about-us/members/united-kingdom/(Accessed August, 2021).
Chapelle, F. H. (2000). Ground-water Microbiology and Geochemistry. New Jersey, US: John Wiley & Sons.
Cl:AIRE, (2021). Good Practice for Risk Assessment for Coal Mine Gas Emissions. CL: AIRE, Buckinghamshire. 978-1-905046-39-3.
Clitherow, B. (2021). Is it Possible to Predict the Formation of Biofilms in Geothermal Systems? MSc thesis. Exeter, England: University of Exeter.
Committee on Climate Change (2016). Next Steps for UK Heat Policy. Available from: https://www.theccc.org.uk/wp-content/uploads/2016/10/Next-steps-for-UK-heat-policy-Committee-on-Climate-Change-October-2016.pdf.
Darling, W. G., Bath, A. H., and Talbot, J. C. (2003). The O and H Stable Isotope Composition of Freshwaters in the British Isles. 2. Surface Waters and Groundwater. Hydrol. Earth Syst. Sci. 7 (2), 183–195. doi:10.5194/hess-7-183-2003
DEFRA (2014). SP1010: Development of Category 4 Screening Levels for Assessment of Land Affected by Contamination – Policy Companion Document. London: Department for Environment. Food and Rural Affairs.
Deichmann, N., and Giardini, D. (2009). Earthquakes Induced by the Stimulation of an Enhanced Geothermal System below Basel (Switzerland). Seismol. Res. Lett. 80 (5), 784–798. doi:10.1785/gssrl.80.5.784
Demski, C. (2021). Net Zero Public Engagement and Participation, Research Note. Department for Business, Energy and Industrial Strategy. Available at: https://assets.publishing.service.gov.uk/government/uploads/system/uploads/attachment_data/file/969428/net-zero-public-engagement-participation-research-note.pdf.
Dickie, J., Watson, E., and Napier, H. (2020). Evaluating the Relationship between Public Perception, Engagement and Attitudes towards Underground Energy Technologies. Report No. OR/20/056. Nottingham, UK: British Geological Survey, 47. Available at: http://nora.nerc.ac.uk/id/eprint/529041/.
Ehrlich, H. L., Newman, D. K., and Kappler, A. (2002). Geomicrobiology. New City, NY: Marcel Dekker.
Farr, G., Busby, J., Wyatt, L., Crooks, J., Schofield, D. I., and Holden, A. (2020). The Temperature of Britain's Coalfields. Q. J. Eng. Geol. Hydrogeology 54, qjegh2020–109. doi:10.1144/qjegh2020-109
Ferretti, A., Fumagalli, A., Novali, F., Prati, C., Rocca, F., and Rucci, A. (2011). A New Algorithm for Processing Interferometric Data-Stacks: SqueeSAR. IEEE Trans. Geosci. Remote Sens. 49 (9), 3460–3470. doi:10.1109/TGRS.2011.2124465
Flude, S., Györe, D., Stuart, F. M., Zurakowska, M., Boyce, A. J., Haszeldine, R. S., et al. (2017). The Inherent Tracer Fingerprint of Captured CO 2. Int. J. Greenh. Gas Control 65, 40–54. doi:10.1016/j.ijggc.2017.08.010
Fordyce, F. M., Brown, S. E., Ander, E. L., Rawlins, B. G., O'Donnell, K. E., Lister, T. R., et al. (2005). GSUE: Urban Geochemical Mapping in Great Britain. Geochem. Explor. Environ. Anal. 5, 325–336. doi:10.1144/1467-7873/05-069
Fordyce, F. M., Everett, P. A., Bearcock, J. M., Lister, T. R., Gowing, C., Watts, M., et al. (2017). Soil Geochemical Atlas of the Clyde Basin. Open Report, OR/14/032. Edinburgh: British Geological Survey. Available at: http://nora.nerc.ac.uk/id/eprint/519195.
Fordyce, F. M., Everett, P. A., Bearcock, J. M., and Lister, T. R. (2017). Soil Metal/metalloid Concentrations in the Clyde Basin, Scotland, UK: Implications for Land Quality. Earth Environ. Sci. Trans. R. Soc. Edinb. 108 (2-3), 191–216. doi:10.1017/S1755691018000282
Fordyce, F. M., Nice, S. E., Lister, T. R., Ó Dochartaigh, B. É., Cooper, R., Allen, M., et al. (2012). Urban Soil Geochemistry of Glasgow. Open Report, OR/08/002. Nottingham, UK: British Geological Survey. Available at: http://nora.nerc.ac.uk/18009/.
Fordyce, F. M., Ó Dochartaigh, B. É., Lister, T. R., Cooper, R., Kim, A., Harrison, I., et al. (2004). Clyde Tributaries: Report of Urban Stream Sediment and Surface Water Geochemistry for Glasgow. Commissioned Report, CR/04/037. Nottingham, UK: British Geological Survey. Available at: http://nora.nerc.ac.uk/18996/.
Fordyce, F. M., Shorter, K. M., Vane, C. H., Gowing, C. J. B., Hamilton, E. M., Kim, A. W., et al. (2020b). UK Geoenergy Observatories GGERFS_SoilChem03-18Data_Release. doi:10.5285/0bfdeb32-db24-4221-9d02-f074f51edff2
Fordyce, F. M., Shorter, K. M., Vane, C. H., Gowing, C. J. B., Hamilton, E. M., Kim, A. W., et al. (2020a). UK Geoenergy Observatories, Glasgow Environmental Baseline Soil Chemistry Dataset. Open Report, OR/19/062. Edinburgh, UK: British Geological Survey, 77. Available at: http://nora.nerc.ac.uk/id/eprint/527798/.
Fordyce, F. M., Shorter, K. M., Walker-Verkuil, K., Barlow, T., Sloane, H. J., Arrowsmith, C., et al. (2021). UK Geoenergy Observatories, Glasgow Environmental Baseline Surface Water Chemistry Dataset 1. Report No. OR/20/061. Edinburgh, UK: British Geological Survey, 122. Available at: http://nora.nerc.ac.uk/id/eprint/529818/.
Frohlich, C., and Pulliam, J. (1999). Single-station Location of Seismic Events: a Review and a Plea for More Research. Phys. Earth Planet. InteriorsEarth Planet. Inter. 113, 277–291. doi:10.1016/s0031-9201(99)00055-2
Gee, D., Bateson, L., Grebby, S., Novellino, A., Sowter, A., Wyatt, L., et al. (2020). Modelling Groundwater Rebound in Recently Abandoned Coalfields Using DInSAR. Remote Sens. Environ. 249, 112021. doi:10.1016/j.rse.2020.112021
Gee, D., Bateson, L., Sowter, A., Grebby, S., Novellino, A., Cigna, F., et al. (2017). Ground Motion in Areas of Abandoned Mining: Application of the Intermittent SBAS (ISBAS) to the Northumberland and Durham Coalfield, UK. Geosciences 7 (3), 85. doi:10.3390/geosciences7030085
Glassley, W. E., Crossey, L. J., and Montañez, I. P. (2016). Fluid-Rock Interaction. Encycl. Geochem., 1–4. doi:10.1007/978-3-319-39193-9_36-1
Gluyas, J. G., Adams, C. A., and Wilson, I. A. G. (2020). The Theoretical Potential for Large-Scale Underground Thermal Energy Storage (UTES) within the UK. Energy Rep. 6, 229–237. doi:10.1016/j.egyr.2020.12.006
Graham, M. T., Ball, D. F., Ó Dochartaigh, B. É., and MacDonald, A. M. (2009). Using Transmissivity, Specific Capacity and Borehole Yield Data to Assess the Productivity of Scottish Aquifers. Q. J. Eng. Geol. Hydrogeology 42, 227–235. doi:10.1144/1470-9236/08-045
Gross, R., and Hanna, R. (2019). Path Dependency in Provision of Domestic Heating. Nat. Energy 4, 358–364. doi:10.1038/s41560-019-0383-5
Halford, K. J., Weight, W. D., and Schreiber, R. P. (2006). Interpretation of Transmissivity Estimates from Single-Well Pumping Aquifer Tests. Ground Water 44, 467–471. doi:10.1111/j.1745-6584.2005.00151.x
Hall, A., Scott, J. A., and Shang, H. (2011). Geothermal Energy Recovery from Underground Mines. Renew. Sustain. Energy Rev. 15, 916–924. doi:10.1016/j.rser.2010.11.007
Hall, J., Glendinning, S., and Younger, P. (2005). “Is Mine Water a Source of Hazardous Gas?,” in 9th INTERNATIONAL MINE WATER CONGRESS, Oviedo, Asturias, Spain, 5-7 September 2005.
Hendry, M. J., Barbour, S. L., Schmeling, E. E., Mundle, S. O. C., and Huang, M. (2016). Fate and Transport of Dissolved Methane and Ethane in Cretaceous Shales of the Williston Basin, Canada. Water Resour. Res. 52, 6440–6450. doi:10.1002/2016WR019047
Hendry, M. J., Schmeling, E. E., Barbour, S. L., Huang, M., and Mundle, S. O. C. (2017). Fate and Transport of Shale-Derived, Biogenic Methane. Sci. Rep. 7, 4881. doi:10.1038/s41598-017-05103-8
HM Government, (2021). Net Zero Strategy: Build Back Greener. Available from: https://assets.publishing.service.gov.uk/government/uploads/system/uploads/attachment_data/file/1033990/net-zero-strategy-beis.pdf.
HM Government, (2020). Powering Our Net Zero Future, Energy White Paper, CP 337. Available from: https://assets.publishing.service.gov.uk/government/uploads/system/uploads/attachment_data/file/945899/201216_BEIS_EWP_Command_Paper_Accessible.pdf.
Huber, S. A., Balz, A., Abert, M., and Pronk, W. (2011). Characterisation of Aquatic Humic and Non-humic Matter with Size-Exclusion Chromatography - Organic Carbon Detection - Organic Nitrogen Detection (LC-OCD-OND). Water Res. 45, 879–885. doi:10.1016/j.watres.2010.09.023
Janson, E., Boyce, A. J., Burnside, N., and Gzyl, G. (2016). Preliminary Investigation on Temperature, Chemistry and Isotopes of Mine Water Pumped in Bytom Geological Basin (USCB Poland) as a Potential Geothermal Energy Source. Int. J. Coal Geol. 164, 104–114. doi:10.1016/j.coal.2016.06.007
Jenkins, C. R., Cook, P. J., Ennis-King, J., Undershultz, J., Boreham, C., Dance, T., et al. (2012). Safe Storage and Effective Monitoring of CO2 in Depleted Gas Fields. Proc. Natl. Acad. Sci. U.S.A. 109 (2), E35–E41. doi:10.1073/pnas.1107255108
Johnson, C. C., Demetriades, A., Locutura, J., and Ottesen, R. T., (Editors) (2011). Mapping the Chemical Environment of Urban Areas (Oxford: Wiley). Minerals (Berlin, Germany: Walter de Gruyter GmbH & Co KG), 8.
Jones, H. K., Morris, B. L., Cheney, C. S., Brewerton, L. J., Merrin, P. D., Lewis, M. A., et al. (2000). The Physical Properties of Minor Aquifers in England and Wales. Technical Report, WD/00/04.. Nottingham, UK: British Geological Survey.
Jordan, C., Bateson, L., and Novellino, A. (2019). Environmental Baseline Monitoring for Shale-Gas Development: Insights for Monitoring Ground Motion Using InSAR Analysis. Sci. Total Environ. 696, 134075. doi:10.1016/j.scitotenv.2019.134075
Kim, A. W., Vane, C. H., Moss-Hayes, V. L., Beriro, D. J., Nathanail, C. P., Fordyce, F. M., et al. (2019). Polycyclic aromatic hydrocarbons (PAH) and polychlorinated biphenyls (PCB) in urban soils of Glasgow, UK. The Geosciences in Europe’s Urban Sustainability: Lessons from Glasgow and Beyond (CUSP). Earth Environ. Sci. Trans. R. Soc. Edinb. 108 (2-3), 231–247. doi:10.1017/S1755691018000324
Kim, H., and Lee, J. Y. (2019). Effects of a groundwater heat pump on thermophilic bacteria activity. WaterSwitzerl. 11 (10). doi:10.3390/w11102084
Lecocq, T., Hicks, S. P., Van Noten, K., van Wijk, K., Koelemeijer, P., De Plaen, R. S. M., et al. (2020). Global quieting of high-frequency seismic noise due to COVID-19 pandemic lockdown measures. Science 369, 1338–1343. doi:10.1126/science.abd2438
Lerm, S., Westphal, A., Miethling-Graff, R., Alawi, M., Seibt, A., Wolfgramm, M., et al. (2013). Thermal effects on microbial composition and microbiologically induced corrosion and mineral precipitation affecting operation of a geothermal plant in a deep saline aquifer. Extremophiles 17 (17), 311–327. doi:10.1007/s00792-013-0518-8
Loredo, C., Ordóñez, A., Garcia-Ordiales, E., Álvarez, R., Roqueñi, N., Cienfuegos, P., et al. (2017). Hydrochemical characterization of a mine water geothermal energy resource in NW Spain. Sci. Total Environ. 576, 59–69. doi:10.1016/j.scitotenv.2016.10.084
Luckett, R. (2021). Earthquake Detection Using Multiple Seismometers in a Single Borehole - A Case Study from the Glasgow Geothermal Energy Research Field Site. Internal Report, IR/21/006. Nottingham, UK: British Geological Survey.
Luek, J. L., and Gonsior, M. (2017). Organic compounds in hydraulic fracturing fluids and wastewaters: A review. Water Res. 123, 536–548. doi:10.1016/j.watres.2017.07.012
Lund, J. W., and Toth, A. N. (2021). Direct utilization of geothermal energy 2020 worldwide review. Geothermics 90, 101915. doi:10.1016/j.geothermics.2020.101915
MacDonald, A. M., Ó Dochartaigh, B. É., and Smedley, P. L. (2017). Baseline Groundwater Chemistry in Scotland’s Aquifers. Open Report, OR/17/030. Nottingham, UK: British Geological Survey, 77. Available at: http://nora.nerc.ac.uk/id/eprint/519084/.
Monaghan, A. A., Damaschke, M., Starcher, V., Fellgett, M. W., Kingdon, A., Kearsey, T., et al. (2021b). UK Geoenergy Observatories Glasgow: GGC01 Cored, Seismic Monitoring Borehole – Final Data Release. Open Report, OR/21/031. Nottingham, UK: British Geological Survey, 64. Available at: http://nora.nerc.ac.uk/id/eprint/530762/.
Monaghan, A. A., Starcher, V., Barron, H. F., Shorter, K., Walker-Verkuil, K., Elsome, J., et al. (2021a). Drilling into mines for heat: geological synthesis of the UK Geoenergy Observatory in Glasgow and implications for mine water heat resources. Q. J. Eng. Geol. Hydrogeology 2021. doi:10.1144/qjegh2021-033
Monaghan, A. A., Starcher, V., Ó Dochartaigh, B. E., Shorter, K. M., and Burkin, J. (2018). UK Geoenergy Observatories: Glasgow Geothermal Energy Research Field Site: Science Infrastructure. Open Report, OR/18/037. Nottingham, UK: British Geological Survey. Available at: http://nora.nerc.ac.uk/id/eprint/521444/.
Nathanail, C. P., McCaffrey, C., Gillett, A. G., Ogden, R. C., and Nathanail, J. F. (2015). The LQM/CIEH S4ULs for Human Health Risk Assessment. Nottingham: Land Quality Press. Copyright Land Quality Management Limited reproduced with permission. Publication Number S4UL3083.
National River Flow Archive (2022). For the River Clyde at Daldowie. Available from: https://nrfa.ceh.ac.uk/data/station/meanflow/84013.
Nelep, (2021). The Case for Mine Energy – unlocking deployment at scale in the UK. A mine energy white paper. Available from: https://www.northeastlep.co.uk/wp-content/uploads/2021/05/Mine-Energy-White-Paper_FINAL.pdf (Accessed December, 2021).
NERC, University of Strathclyde and BGS (2019). Record of proceedings UK Geoenergy Observatories Glasgow Geothermal Energy Innovation Workshop. https://cms.ukgeos.ac.uk/event/assets/innovation_event_030619_final.pdf (Accessed November, 2020).
Novellino, A., Bateson, L., and Jordan, C. (2021). Ground motion baseline analysis of the Cheshire UK GeoEnergy Observatory. Sci. Rep. 11 (1), 1–11. doi:10.1038/s41598-021-95191-4
Ó Dochartaigh, B. E., Bonsor, H., and Bricker, S. (2019). Improving understanding of shallow urban groundwater: The Quaternary groundwater system in Glasgow, UK. Earth Environ. Sci. Trans. R. Soc. Edinb. 108, 155–172. doi:10.1017/S1755691018000385
Ó Dochartaigh, B. E., MacDonald, A. M., Fitzsimons, V., and Ward, R. (2015). Scotland’s aquifers and groundwater bodies. Open Report OR/015/028. Nottingham, UK: British Geological Survey, 63. Available at: http://nora.nerc.ac.uk/id/eprint/511413/.
Optimat (2019). Glasgow Geothermal Energy Research Field Site Company demand analysis. https://www.evaluationsonline.org.uk/evaluations/Search.do?ui=basic&action=show&id=693 (Accessed February 4, 2022).
Orem, W., Tatu, C., Varonka, M., Lerch, H., Bates, A., Engle, M., et al. (2014). Organic substances in produced and formation water from unconventional natural gas extraction in coal and shale. Int. J. Coal Geol. 126 (20)–31. doi:10.1016/j.coal.2014.01.003
Osvald, M., Maróti, G., Pap, B., and Szanyi, J. (2017). Biofilm Forming Bacteria during Thermal Water Reinjection. Geofluids 22, 1–7. doi:10.1155/2017/9231056
Palumbo-Roe, B., Banks, V. J., Bonsor, H. C., Hamilton, E. M., and Watts, M. J. (2017). Limitations on the role of the hyporheic zone in chromium natural attenuation in a contaminated urban stream. Appl. Geochem. 83, 108–120.
Palumbo-Roe, B., Shorter, K. M., Fordyce, F. M., Walker-Verkuil, K., Ó Dochartaigh, B. E., Gooddy, D. C., et al. (2021). UK Geoenergy Observatories: Glasgow Borehole Test Pumping - Groundwater Chemistry. Open Report, OR/21/030. Nottingham, UK: British Geological Survey Open Report, 73. Available at: http://nora.nerc.ac.uk/id/eprint/531098/.
Preene, M., and Younger, P. L. (2014). Can you take the heat? – Geotherm. energy Min. Min. Technol. 123 (2), 107–118. doi:10.1179/1743286314Y.0000000058
Ramos, E. P., Breede, K., and Falcone, G. (2015). Geothermal heat recovery from abandoned mines: a systematic review of projects implemented worldwide and a methodology for screening new projects. Environ. Earth Sci. 73, 6783–6795. doi:10.1007/s12665-015-4285-y
Ramsay, C., McRae, C., Ryan, E., McCallum, A., Wellington, L., Lauder, L., et al. (2017). Carbon dioxide ingress into residential houses at Gorebridge in Midlothian, Scotland, United Kingdom Richard Othieno. Eur. J. Public Health 27 (Suppl. l_3). doi:10.1093/eurpub/ckx187.278
REA/Arup (2021). Deep Geothermal Energy. Economic Decarbonisation Opportunities for the United Kingdom. Available from: https://www.r-e-a.net/wp-content/uploads/2021/05/Deep-Geothermal-Energy-Opportunities-for-the-UK.pdf.
Ren21, (2021). Key messages for decision makers: takeaways from the renewables 2021 global status report. Available from: https://www.ren21.net/wp-content/uploads/2019/05/GSR2021_Key_Messages.pdf.
Romanak, K. D., Bennett, P. C., Yang, C., and Hovorka, S. D. (2012). Process-based approach to CO2 leakage detection by vadose zone gas monitoring at geologic CO2 storage sites. Geophys. Res. Lett. 39, L15405. doi:10.1029/2012GL052426
Sadeghi, Z., Wright, T. J., Hooper, A. J., Jordan, C., Novellino, A., Bateson, L., et al. (2021). Benchmarking and Inter-Comparison of Sentinel-1 InSAR velocities and time series. Remote Sens. Environ. 256, 112306. doi:10.1016/j.rse.2021.112306
Sánchez-Vila, X., Meier, P. M., and Carrera, J. (1999). Pumping tests in heterogeneous aquifers: An analytical study of what can be obtained from their interpretation using Jacob's Method. Water Resour. Res. 35, 943–952. doi:10.1029/1999WR900007
SEPA, (2019). Environmental Quality Standards and Standards for Discharges to Surface Waters. Supporting Guidance (WAT-SG-53). Stirling: Scottish Environment Protection Agency.
SEPA, (2014). The Scotland River Basin District (Standards) Directions 2014. Stirling: Scottish Environment Protection Agency.
Shorter, K. M., Palumbo-Roe, B., Fordyce, F. M., O Dochartaigh, B. E., and Walker-Verkuil, K. (2021b). UK Geoenergy Observatories Glasgow : groundwater chemistry data collected during the borehole construction phase. Open report OR/21/015. Edinburgh, UK: British Geological Survey, 45. Available at: http://nora.nerc.ac.uk/id/eprint/530443/.
Shorter, K., O Dochartaigh, B. E., Butcher, A., MacDonald, A., Elsome, K., and Burke, S. (2021a). Data release and initial interpretation of test pumping of boreholes at the Glasgow UK Geoenergy Observatory. Open Report, OR/21/016. Nottingham, UK: British Geological Survey. Available at: http://nora.nerc.ac.uk/id/eprint/530507/.
Simioni, M., Gavey, R., and Wang, X. (2021). Low carbon subsurface technologies: identifying potential environmental impacts. https://assets.publishing.service.gov.uk/government/uploads/system/uploads/attachment_data/file/1026921/Low_carbon_subsurface_technologies_-_identifying_potential_environmental_impacts_-_report.pdf.
Smedley, P. L., Bearcock, J. M., Fordyce, F. M., Everett, P. A., Chenery, S., and Ellen, R. (2017). Stream Water Geochemical Atlas of the Clyde Basin. Open Report OR/16/015. Nottingham, UK: British Geological Survey. Available at: http://nora.nerc.ac.uk/id/eprint/519332.
Smith, D., Taylor-Curran, H., Barkwith, A., Lister, T. R., Kirk, K., Hannis, S., et al. (2021). Applying ground gas and gas flux monitoring techniques to low-enthalpy, shallow geothermal energy exploration. Geothermics 97. doi:10.1016/j.geothermics.2021.102251
Smith, E. D., and Liu, X. (2018). Evaluation of the Impacts of Heat Exchanger Operation on Quality of Water Used as Heat Source and Sink. U.S. Department of Energy Office of Scientific and Technical Information, 13–19.
Sowter, A., Bateson, L., Strange, P., Ambrose, K., and Syafiudin, M. F. (2013). DInSAR estimation of land motion using intermittent coherence with application to the South Derbyshire and Leicestershire coalfields. Remote Sens. Lett. 4, 979–987. doi:10.1080/2150704X.2013.823673
Starcher, V., Monaghan, A. A., Barron, H. F., Shorter, K., Walker-Verkuil, K., and Elsome, J. (2021). Method and key observations from constructing a mine water heat subsurface observatory in Glasgow UK. Open Report, OR/21/020. Nottingham, UK: British Geological Survey. Available at: http://nora.nerc.ac.uk/id/eprint/530822/.
Stephenson, M. H., Ringrose, P., Geiger, S., Bridden, M., and Schofield, D. (2019). Geoscience and decarbonization: current status and future directions. Pet. Geosci. 25, 501–508. doi:10.1144/petgeo2019-084
Steven, J. (2021). “From Venture Pit to Walker Shore, coal and heat and fathoms of core’: Mine water Heat Exploitation in Newcastle/Gateshead,” in Presentation Accessed August 2021 at IEA Geothermal | 2021 Mine Water Geothermal Energy Symposium - International Energy Agency Geothermal (Department for Business, Energy and Industrial Strategy).
The Coal Authority (2020). Geothermal energy from abandoned coal mines. https://www2.groundstability.com/geothermal-energy-from-abandoned-coal-mines/(accessed November, 2020).
The Coal Authority (2019). Guidance on Managing the Risk of Hazardous Gases when Drilling or Piling Near Coal: Version 2. Available from: https://assets.publishing.service.gov.uk/government/uploads/system/uploads/attachment_data/file/810431/Guidance_on_managing_the_risk_of_hazardous_gases_when_drilling_or_piling_near_coal.pdf.
Thouvenot, F., and Bouchon, M. (2008). “What is the Lowest Magnitude Threshold at Which an Earthquake can be Felt or Heard, or Objects Thrown into the Air?,” in Historical Seismology. Modern Approaches in Solid Earth Sciences. Editors J. Fréchet, M. Meghraoui, and M. Stucchi (Dordrecht: Springer). doi:10.1007/978-1-4020-8222-1_15
Todd, F., McDermott, C., Fraser Harris, A., Bond, A., and Gilfillan, S. (2019). Coupled hydraulic and mechanical model of surface uplift due to mine water rebound: implications for mine water heating and cooling schemes. Scott. J. Geol. 55, 124–133. doi:10.1144/sjg2018-028
Townsend, D. H., Naismith, J. D. A., Townsend, P. J., Milner, M. G., and Fraser, U. T. (2021). “On the Rocks – Exploring Business Models for Geothermal Heat in the Land of Scotch,” in Proceedings World Geothermal Congress 2020, Reykjavik, Iceland, April 26–May 2, 2020.
Tyler, J. J., Jones, M., Arrowsmith, C., Allot, T., and Leng, M. J. (2016). Spatial patterns in the oxygen isotope composition of daily rainfall in the British Isles. Clim. Dyn. 47, 1971–1987. doi:10.1007/s00382-015-2945-y
Uktag, (2013). Updated Recommendations on Phosphorus Standards for Rivers (2015 – 2025). London: UK Technical Advisory Group on the Water Framework Directive.
Verhoeven, R., Willems, E., Harcouët-Menou, V., De Boever, E., Hiddes, L., Op’T Veld, P., et al. (2014). Minewater 2.0 project in Heerlen the Netherlands: transformation of a geothermal mine water pilot project into a full scale hybrid sustainable energy infrastructure for heating and cooling. Energy Procedia 46, 58–67. doi:10.1016/j.egypro.2014.01.158
Vieth, A., Mangelsdorf, K., Sykes, R., and Horsfield, B. (2008). Water extraction of coals - potential to estimate low molecular weight organic acids as carbon feedstock for the deep terrestrial biosphere. Org. Geochem. 39, 985–991. doi:10.1016/j.orggeochem.2008.02.012
VROM (2009). Circular on Target Values and Intervention Values for Soil Remediation. The Hague: Ministry of Housing, Spatial Planning and Environment.
Walls, D. B., Banks, D., Boyce, A. J., and Burnside, N. M. (2021). A review of the performance of minewater heating and cooling systems. Energies 14, 6215. doi:10.3390/en14196215
Ward, R. S., Smedley, P. L., Allen, G., Baptie, B. J., Barkwith, A. K. A. P., Bateson, L., et al. (2019). Environmental monitoring - phase 4 final report (April 2018 - March 2019). Open Report, OR/19/044. Nottingham, UK: British Geological Survey, 225. Available at: http://nora.nerc.ac.uk/id/eprint/527726/.
Watson, S. D., Lomas, K. J., and Buswell, R. A. (2019). Decarbonising domestic heating: What is the peak GB demand? Energy Policy 126, 533–544. doi:10.1016/j.enpol.2018.11.001
Watson, S., and Westaway, R. (2020). Borehole temperature log from the Glasgow Geothermal Energy Research Field Site: a record of past changes to ground surface temperature caused by urban development. Scott. J. Geol. 6, 134–152. doi:10.1144/sjg2019-033
Watzlaf, G. R., and Ackman, T. E. (2006). Underground mine water for heating and cooling using geothermal heat pump systems. Mine Water Environ. 25, 1–14. doi:10.1007/s10230-006-0103-9
Wilke, F. D. H., Vieth-Hillebrand, A., Naumann, R., and Jörg Erzinger, B. H. (2015). Induced mobility of inorganic and organic solutes from black shales using water extraction: Implications for shale gas exploitation. Appl. Geochem. 63, 158–168. doi:10.1016/j.apgeochem.2015.07.008
Williams, J. D., Dobbs, M. R., Kingdon, A., Lark, R. M., Williamson, J. P., MacDonald, A. M., et al. (2017). Stochastic modelling of hydraulic conductivity derived from geotechnical data; an example applied to central Glasgow. Earth Environ. Sci. Trans. R. Soc. Edinb. 108, 141–154. doi:10.1017/S1755691018000312
Wördemann, H., Westphal, A., Lerm, S., Kleyböcker, A., Teitz, S., Kasina, M., et al. (2014). Influence of microbial processes on the operational reliability in a geothermal heat store - Results of long-term monitoring at a full scale plant and first studies in a bypass system. Energy Procedia 59, 412–417.
Younger, P. L. (2001). Mine water pollution in Scotland: nature, extent and preventative strategies. Sci. Total Environ. 265, 309–326. doi:10.1016/s0048-9697(00)00673-2
Keywords: geochemistry, geothermal, mine water, environmental monitoring, geomicrobiology
Citation: Monaghan AA, Bateson L, Boyce AJ, Burnside NM, Chambers R, de Rezende JR, Dunnet E, Everett PA, Gilfillan SMV, Jibrin MS, Johnson G, Luckett R, MacAllister DJ, MacDonald AM, Moreau JW, Newsome L, Novellino A, Palumbo-Roe B, Pereira R, Smith D, Spence MJ, Starcher V, Taylor-Curran H, Vane CH, Wagner T and Walls DB (2022) Time Zero for Net Zero: A Coal Mine Baseline for Decarbonising Heat. Earth Sci. Syst. Soc. 2:10054. doi: 10.3389/esss.2022.10054
Received: 02 March 2022; Accepted: 15 June 2022;
Published: 12 August 2022.
Edited by:
Jennifer J. Roberts, University of Strathclyde, United KingdomReviewed by:
Charlotte Adams, Coal Authority, United KingdomAaron Cahill, Heriot-Watt University, United Kingdom
British Geological Survey © UKRI 2022. This is an open-access article distributed under the terms of the Creative Commons Attribution License (CC BY). The use, distribution or reproduction in other forums is permitted, provided the original author(s) and the copyright owner(s) are credited and that the original publication in this journal is cited, in accordance with accepted academic practice. No use, distribution or reproduction is permitted which does not comply with these terms.
*Correspondence: Alison A. Monaghan, YWxzQGJncy5hYy51aw==