- 1School of GeoSciences, Grant Institute, University of Edinburgh, Edinburgh, United Kingdom
- 2Department of Civil and Environmental Engineering, University of Strathclyde, Glasgow, United Kingdom
- 3Isotope Geosciences Unit, Scottish Universities Environmental Research Centre (SUERC), East Kilbride, United Kingdom
Usage of thermal energy contained in abandoned, flooded, coal mines has the potential to contribute to low carbon heating or cooling supply and assist in meeting net-zero carbon emission targets. However, hazardous ground gases, such as CH4 and CO2, can be found naturally in superficial deposits, coal bearing strata and abandoned mines. Determining the presence, magnitude, and origin of subsurface gases, and how their geochemical fingerprints evolve within the shallow subsurface is vital to developing an understanding of how to manage the risk posed by ground gases in geoenergy technology development. Here, we present the first CH4 and CO2 concentration-depth profiles and stable isotope (δ13CCH4, δ13CCO2, and δDCH4) profiles obtained from UK mine workings, through analysis of headspace gas samples degassed from cores and chippings collected during construction of the Glasgow Observatory. These are used to investigate the variability of gas fingerprints with depth within unmined Carboniferous coal measures and Glasgow coal mine workings. Stable isotope compositions of CH4 (δ13CCH4 = −73.4‰ to −14.3‰; δ13CCO2 = −29‰ to −6.1‰; δDCH4 = −277‰ to −88‰) provide evidence of a biogenic source, with carbonate reduction being the primary pathway of CH4 production. Gas samples collected at depths of 63–79 m exhibit enrichments in 13CCH4 and 2H, indicating the oxidative consumption of CH4. This correlates with their proximity to the Glasgow Ell mine workings, which will have increased exposure to O2 from the atmosphere as a result of mining activities. CO2 gas is more abundant than CH4 throughout the succession in all three boreholes, exhibiting high δ13CCO2 values relative to the CH4 present. Gases from unmined bedrock exhibit the highest δ13CCO2 values, with samples from near-surface superficial deposits having the lowest δ13CCO2 values. δ13CCO2 values become progressively lower at shallower depths (above 90 m), which can be explained by the increasing influence of shallow groundwaters containing a mixture of dissolved marine carbonate minerals (∼0‰) and soil gas CO2 (−26‰) as depth decreases. Our findings provide an insight into the variability of mine derived gases within 200 m of the surface, providing an important ‘time-zero’ record of the site, which is required in the design of monitoring approaches.
Introduction
The use of thermal energy contained within groundwater in abandoned, flooded, coal mines has considerable potential to contribute to the provision of low carbon heating or cooling to assist in meeting global net-zero carbon emission targets (Adams et al., 2019; Stephenson et al., 2019; Monaghan et al., 2022a). A quarter of UK homes and businesses lie above former coalfields, providing a highly permeable network of buried mine workings flooded with water at above-ambient temperatures (Adams et al., 2019; Monaghan et al., 2022b). However, there are a multitude of manageable, but significant techno-societal risks associated with the utilisation of the heat from minewaters, related to both the direct site operation and the environment surrounding it, such as resource sustainability and efficiency, reservoir quality, operation maintenance, ground motion, ground gases and environmental change (University of Strathclyde, and BGS, 2019; NERC, University of Strathclyde and BGS, 2019; Monaghan et al., 2022a).
In order to address these issues, there is a clear need for applied research on minewater heat utilisation, to provide an open evidence base to enable knowledge transfer to assist with social acceptance, constraining the economic models and reducing development, operational and post closure risk of a mine water heat site (NERC et al., 2019; Stephenson et al., 2019). In conjunction with a growing number of underground laboratories worldwide, the UK Geoenergy Observatory in Glasgow (“Glasgow Observatory”) is a unique facility for investigating shallow, low-temperature mine water thermal energy resources in abandoned and flooded workings at depths of around 50–85 m. This site provides a vital record of the “time-zero” baseline conditions prior to activities commencing at the site and a record of any environmental changes induced by operations to extract or reinject heat into the mine workings.
Coal derived gas is an important energy resource and a potential source of greenhouse gas, as the majority of coal and coal bearing strata contain significant quantities of gases (Hall et al., 2005; CL-AIRE, 2021). These gases pose a significant potential hazard as they are either potentially explosive in critical concentrations when mixed with air, or are toxic to life at elevated (from ambient) concentrations. Gases found in a mine are typically mixtures of atmospheric air, inert gases, water vapour and one or more of the following: O2, CO, CO2, CH4, H2S, H2 and NOx (Hall et al., 2005; CL-AIRE, 2021). Whilst these pose no threat provided they stay in the mine, they can migrate through voids and strata and be emitted at the surface above the mine. Release of this gas as a result of minewater heat extraction would pose both an unwelcome climate feedback of greenhouse gases, and a potential hazard to the local population, as exemplified by recent demolition of a public housing estate in the Scottish town of Gorebridge due to mine gas ingress (Ramsey et al., 2017).
Whilst CH4 associated with coal is predominantly considered as being produced thermogenically due to the burial and thermal maturation of coals, a number of studies have shown that bacterial coal bed CH4 can be produced from microbial activity within lower maturity coals under anoxic conditions (Krüger et al., 2008; Strąpoć et al., 2011; Guo et al., 2012; Gründger et al., 2015). Traditionally, hydrocarbon abundances (C1/(C2+C3) and stable isotopes (δ13CCH4, δ13CCO2, and δDCH4) of CH4 and other associated hydrocarbon gases are used to distinguish between thermogenic and bacterial CH4 sources (Schoell, 1980; Whiticar, 1999; Osborn et al., 2011; Stuart, 2012; Jackson et al., 2013; Györe et al., 2018). Hydrocarbon ratios of 103 to 105, δ13CCH4 of < −55‰; and δ2HCH4 < −150‰ are characteristic of bacterial CH4 (Schoell, 1980); with thermogenic CH4 gas typically containing ratios of <100, with δ13CCH4 values −45‰ to −110‰ and δ2HCH4 > −255‰, respectively (Stuart, 2012; LeDoux et al., 2016). However, several processes can alter the hydrocarbon abundance and stable isotope signature of CH4 and can result in the misidentification of the gas source. Processes include the mixing of different sources of CH4; or microbial oxidation, which can enrich bacterial CH4 in 13C and 2H to that of thermogenic sources (Barker and Fritz, 1981; Whiticar, 1999; Molofsky et al., 2013; LeDoux et al., 2016).
Here, we outline how sampling and analysis of gases from drilling at the Glasgow Observatory during its construction has enabled the determination of the presence, source and volume of coal and mine derived gases (CO2 and CH4) in the subsurface at the site. We use the geochemical tools outlined above to determine the source of the gases encountered and to provide a unique insight into the variation of gas signatures with depth and mining activities within flooded coal mines.
Setting of the UK Geoenergy Observatory in Glasgow, Scotland
The Glasgow Observatory has been developed to investigate the potential energy resource available and variability of low temperature geothermal energy from shallow mine workings (Monaghan et al., 2019). The Observatory is located on the west side of the Central Coalfield of the Midland Valley of Scotland, in the east of the city of Glasgow within the Cuningar Loop (Monaghan et al., 2019) in an area where prolific coal mining activity has occurred. Due to historic coal mining and extensive industrial activity, the site contains significant made ground of waste building material, which overlies Quaternary glacial and post glacial deposits that are up to 25 m thick (Monaghan et al., 2019). These superficial deposits overlie the Scottish Coal Measures Group, a group of fluvio-deltaic Carboniferous sedimentary rocks that contain cyclical sequences of mudstone, siltstone, sandstone, and coals that were deposited during repeated marine regressions and transgressions in the Westphalian period (Cameron and Stephenson, 1985; Monaghan et al., 2019). The Glasgow Observatory’s infrastructure consists of 12 boreholes: a 200 m seismic monitoring borehole (GGC01), drilled and installed on site during a 3-month period from November 2018 to January 2019; and 11 shallow (max 90 m depth) mine characterisation and monitoring boreholes, drilled and installed from May 2019 to January 2020. Superficial deposits and the bedrock encountered by all boreholes at the Cuningar Loop site were drilled by reverse circulation rotary drilling to ensure good sample recovery (Monaghan et al., 2022b). The 11 monitoring boreholes are situated in the Cuningar loop of the River Clyde, on four sub-sites (GGERFS01, GGERFS02, GGERFS03, GGERFS05); with the seismic monitoring borehole located on sub-site GGERFS10, >1.5 km east in the area of Dalmarnock (Figure 1). Strata at site GGERFS10 was unmined, and a continuous 199 m long core was recovered from drilling. All other 11 monitoring boreholes on site encountered shallow mine workings, and rock chipping samples were obtained during drilling.
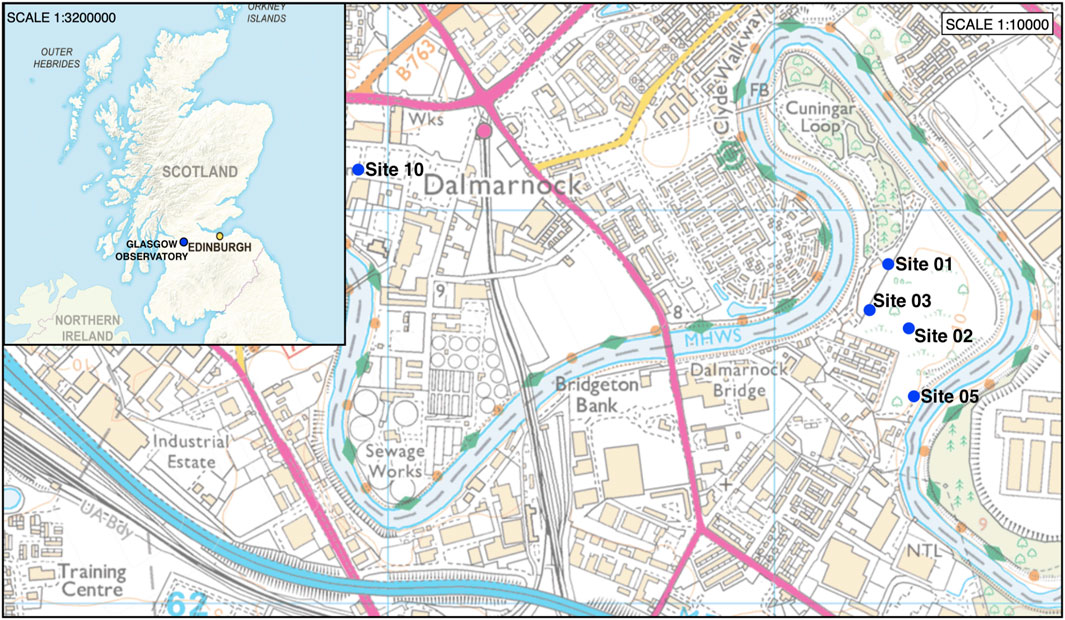
FIGURE 1. The UK Geoenergy Observatory is located in the Eastern side of Glasgow, the largest city in Scotland, located next to the River Clyde, in the Midland Valley of Scotland. The site consists of 12 boreholes, located at five sites, four of these are located within the Cuningar loop formed by a meander of the River Clyde, with the GGC01 borehole located at site 10 in the Dalmarnock area. Contains Ordnance Survey data © Crown copyright and database rights. All rights reserved [2021] Ordnance Survey (100025252).
Hydrogeologically, the glacio-fluvial superficial deposits found on the site are thought to form part of a linear shallow aquifer system, which is up to 2–3 km wide, located beneath Glasgow (Ó Dochartaigh et al., 2019). The superficial aquifer is thought to be highly heterogeneous and complex, due to the heterogeneity of the deposits, and the effect of urban influences (Ó Dochartaigh et al., 2019). The Carboniferous bedrock on the GGERFS site typically forms complex, layered aquifer systems that are dominated primarily by fracture flow (Ó Dochartaigh et al., 2019). Mining of such deposits has resulted in significant changes in the natural groundwater flow paths and hydrogeological conditions (Ó Dochartaigh et al., 2019). The presence of mine voids, workings and other waste materials frequently results in significant change (often increases) in transmissivity within the aquifer, resulting in previously unconnected groundwater bodies to be linked (Ó Dochartaigh et al., 2019; Younger and Robins, 2002).
Materials and Methods
Samples were collected from three boreholes on site: GGA05, located at site GGERFS02; GGA08, located at site GGERFS03; both of which are mine characterisation and monitoring boreholes, and GGC01; the 200 m deep seismic monitoring borehole located in Dalmarnock. (Full borehole data obtained from Monaghan et al. (2021), British Geological Survey (2020a), and British Geological Survey (2020b).
Rock samples consisting of two 50 mm quarter sections of core were obtained approximately every 10 m depth during drilling of the GGC01 seismic monitoring borehole and drill cutting samples from GGA05 and GGA08 boreholes were collected over 3 m depth intervals (Figure 2). The collection of core and cutting samples at 10 and 3 m intervals within the subsurface allowed for additional resolution in the complexity of the gas signatures on site, which would not have been obtained from standard borehole samples. These core and cutting samples were then stored in gas tight isojars prior to analysis of the exsolved gases. Duplicate sampling from the seismic monitoring borehole allowed two different isojar storage methods to be tested; with one set of samples stored in de-ionised water that had 1 mL (20 drops) of Benzalkonium (Zephiran) Chloride biocide added to the Isojar, and the other purged with N2 gas. Preliminary analysis of the samples from the seismic monitoring borehole clearly indicated that storage in de-ionised water resulted in higher concentrations of the exsolved gases, indicating better sample preservation, hence the subsequent obtained cutting samples were solely stored in de-ionised water, with added Benzalkonium (Zephiran) Chloride biocide. The full suite of GC data for all core and cutting samples from both preservation methods is provided in Supplementary Tables S1, S2. All samples were then stored at standard temperature (25°C) and pressure (1atm) for a 2-month period, to allow the samples to equilibrate with the headspace prior to gas analysis conducted at the Scottish Universities Environmental Research Centre (SUERC).
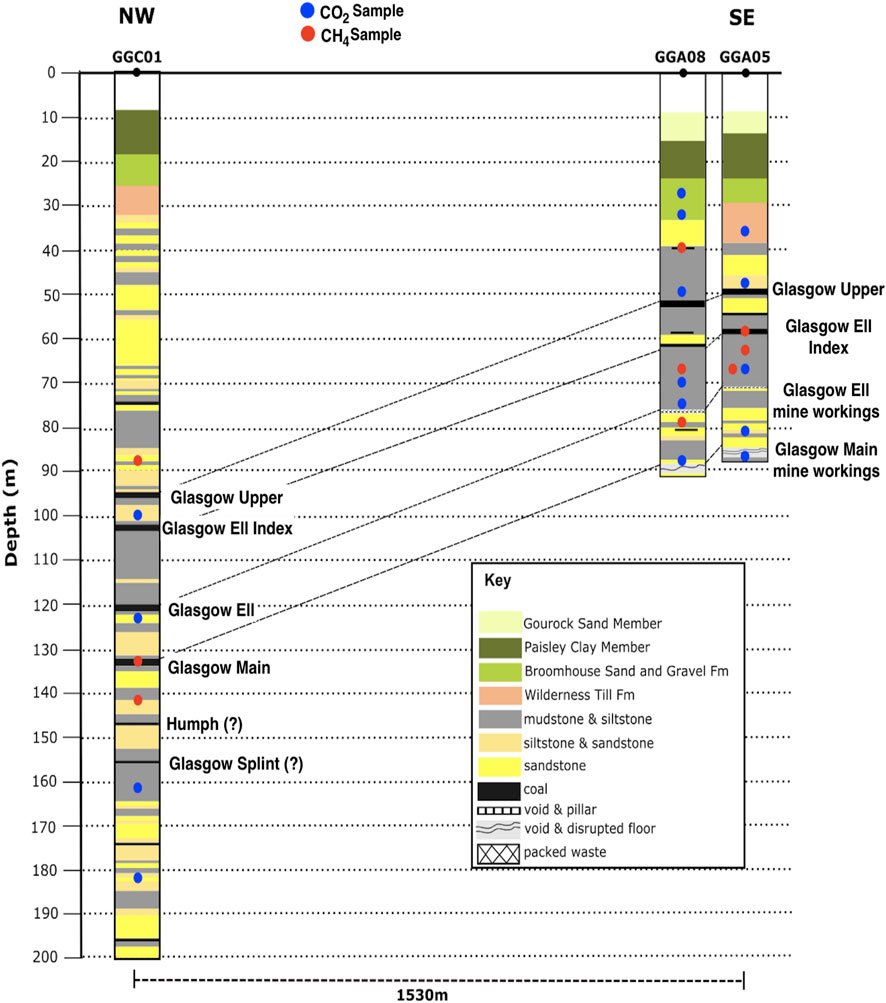
FIGURE 2. Composite logs of GGC01, GGA05, and GGA08 boreholes, and the depths of the core and cutting samples that were obtained for stable isotope analysis. The borehole logs indicate the major coal seams (Glasgow Upper, Glasgow Ell Index, Glasgow Ell, Glasgow Main), with Glasgow Ell and Glasgow Main coal seams have been mined in the shallow GGA05 and GGA08 boreholes. These seams can be correlated to unmined coal seams in GGC01.
50 μL of the gas headspace was collected from the isojars in a 100 μL syringe and injected manually into the septa port of a Perkin-Elmer AutoSystem XL gas chromatograph (GC), via a 30 m long and 0.53 mm internal diameter Sigma-Aldrich Carboxen 1010 PLOT column using helium carrier gas. The GC was also equipped with a flame ionization detector to measure light hydrocarbons and was calibrated with appropriate gas mixtures produced by CalGaz Ltd. Concentration data is recorded as mg/L in the gas phase, as determined from % components, with the full GC data provided in Supplementary Tables S1, S2.
Samples that exhibited CO2 and CH4 concentrations above 1.5% by volume were then selected for stable isotope analysis. Stable isotope determinations were conducted on the gas combustion line at SUERC. The extraction inlet was attached directly to the sealed isojars; with a pressure gradient applied to draw gas through the line. CO2 was separated from volatile hydrocarbons using a procedure modified from Kusakabe (2005). A liquid N2 cooled isopentane trap (−160°C) was applied to collect CO2 and water before an acetone slush bath was used (∼-78°C) to retain water and vaporise CO2. The CO2 was then collected separately in a liquid N2 cooled cold finger. The CH4 samples were combusted over a CuO catalyst at 900°C into CO2 and water, which were collected in a liquid N2 cooled cold finger. A pressure gradient drawing gases through the furnace was maintained by the cold finger trapping combustion products. After combustion, the cold finger was heated with an acetone slush bath (∼−78°C) to retain water and vaporise CO2. This CO2 was collected separately in a separate liquid N2 cooled cold finger. Both the original and combusted CO2 were analysed on a VG SIRA II dual-inlet IRMS, calibrated to internal standards (Dunbar et al., 2016), with measured values relative to V-PDB standards. The cold finger containing the collected water was connected to a manifold, heated to vapour, and reduced to H2 over a nickel catalyst at 800°C. H2 was analysed in a separate Delta Optima Plus dual-inlet IRMS, and calibrated to internal standards (Donnelly et al., 2001). δ13C values are reported relative to V-PDB international standard and δD values are quoted relative to V-SMOW (Craig, 1957; Gonfiantini, 1984; Coplen, 1995) with known uncertainties of 0.3% (δ13C) and 3% (δD).
Results
CH4 and CO2 Gas Concentrations From Core and Cutting Samples
Gas concentration data for all core and cutting samples are provided in Supplementary Tables S1, S2. Exsolved gas headspace analysis of core samples from the unmined GGC01 borehole determined the presence of both CH4 and CO2 gas from depths below 77 m. CH4 gas concentrations for GGC01 range from 6 to 88 mg/L (mean = 17 mg/L, Std. dev = 23 mg/L), with samples with increased concentrations correlating to areas immediately surrounding unmined coal seams (Glasgow Main coal, and potentially the Humph coal and Glasgow Splint coals) (Figure 3). CO2 concentrations in GGC01 occur in samples where CH4 concentrations are lowest or absent, and range from 2 to 118 mg/L (mean = 33 mg/L, Std. dev = 37 mg/L) (Figure 3).
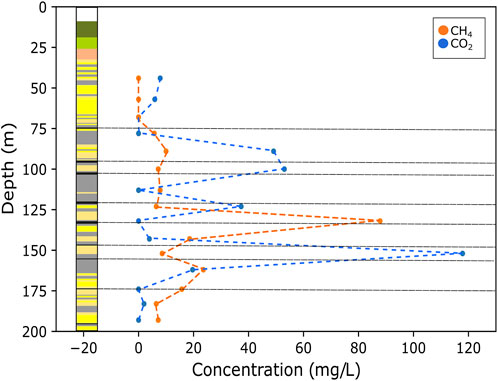
FIGURE 3. Stratigraphic log of BH GGC01 and CH4 and CO2 concentrations with depth (black dashed lines indicate coal seams). The figure highlights that increased concentrations of CH4 gas correlate to areas immediately surrounding the unmined coal seams in the subsurface. The highest CO2 concentrations occurred in samples with lowest CH4 concentrations, or where CH4 was absent.
For mined boreholes GGA05 and GGA08, considerably less instances of elevated CH4 concentrations were found to be present throughout the succession. It is noted that the majority of samples have CH4 levels below detection limits, which compliments groundwater concentration data (Palumbo-Roe et al., 2021) (Figures 4, 5). In GGA08, CH4 gas was identified at four stratigraphic depths, and correlates with unmined coal seams (a minor coal unit at 38–40 m depth, and the Glasgow Upper coal seam at 52–53 m depth), and the area directly below the Glasgow Ell coal mine workings (78–79 m depth). In GGA05, CH4 gas was solely detected at 57–67 m depth in a cluster of samples in the area directly above the collapsed Glasgow Ell mine workings (Figure 4). CH4 concentrations for GGA05 and GGA08 boreholes ranged from 6 to 324 mg/L mean = 53 mg/L, Std. dev = 102 mg/L), with the highest CH4 concentration correlating to the unmined Glasgow Ell index coal seam in GGA05. These values are higher than in-situ groundwater CH4 concentrations recorded, e.g., Glasgow Main (174–185 μg/L) and Glasgow Upper (117–145 μg/L) (Palumbo-Roe et al., 2021).
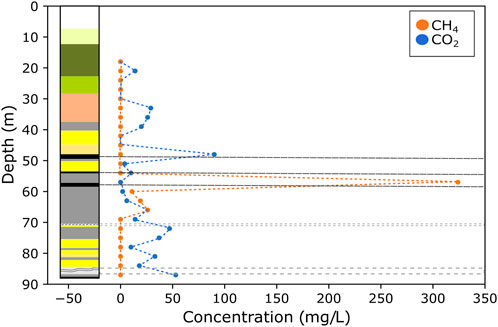
FIGURE 4. Stratigraphic log of BH GGA05 and CH4 and CO2 concentrations with depth (black dashed lines indicate coal seams and grey dashed boxes indicate coal mine workings). CH4 was solely detected at 57–67 m depth in a cluster of samples, in the succession directly above the Glasgow Ell mine workings. The CO2 gas did not show the same trend, and was present throughout the stratigraphic succession.
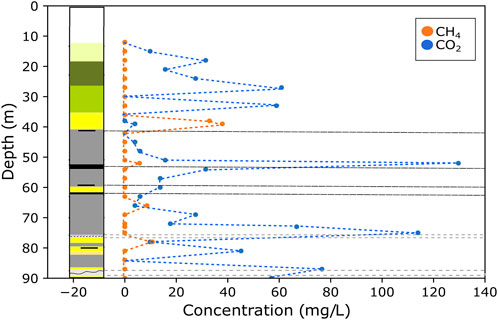
FIGURE 5. Stratigraphic log of BH GGA08 and CH4 and CO2 concentrations with depth black dashed lines indicate coal seams and grey dashed boxes indicate coal mine workings). CH4 was identified at four stratigraphic depths; all of which correspond to areas of coal seams or mine workings. Conversely, CO2 was present throughout the entire stratigraphic sequence, and generally present in higher concentrations than CH4.
However, CO2 gas was present throughout the succession of both GGA05 and GGA08 boreholes, with concentrations ranging from 4 to 130 mg/L (mean = 31 mg/L, Std. dev = 30 mg/L) (Figures 4, 5), and corresponds well with measured groundwater concentrations of 105–256 mg/L (Palumbo-Roe et al., 2021). In both GGA05 and GGA08, the highest CO2 gas concentrations occurred at the unmined Glasgow Upper coal seam, and at both the Glasgow Ell and Glasgow Main mine workings.
CH4 and CO2 Stable Isotope Values
Core samples from the unmined GGC01 borehole exhibit a narrow δ13CCH4 range of −73.4‰ to −64‰, which is characteristic of a biogenic CH4 source (Schoell, 1980; Whiticar et al., 1999; Osborn et al., 2011; Stolper et al., 2018). Associated deuterium values for GGC01 also fall within a narrow range of δDCH4, with values of −277‰ to −240‰, and compliment the biogenic origin implied by δ13CCH4 values. The shallow mined GGA05 and GGA08 boreholes have δ13CCH4 values that range from −70‰ to −14.3‰, and −74.1‰ to −19.7‰, respectively. GGA05 and GGA08 δDCH4 values also exhibit a large range; with values of −182‰ to 17.3‰, and −259‰ to −88‰. However, as evidenced in Table 1, there are four samples from GGA05 and GGA08 that are enriched in 13CCH4 and 2HCH4, which account for the large range in δ13CCH4 and δDCH4 values. Excluding these samples, GGA05 and GGA08 have δ13CCH4 values of −74.1‰ to −70‰ and δDCH4 values of −259‰ to −182‰ corresponding with the biogenic CH4. signatures observed in GGC01.
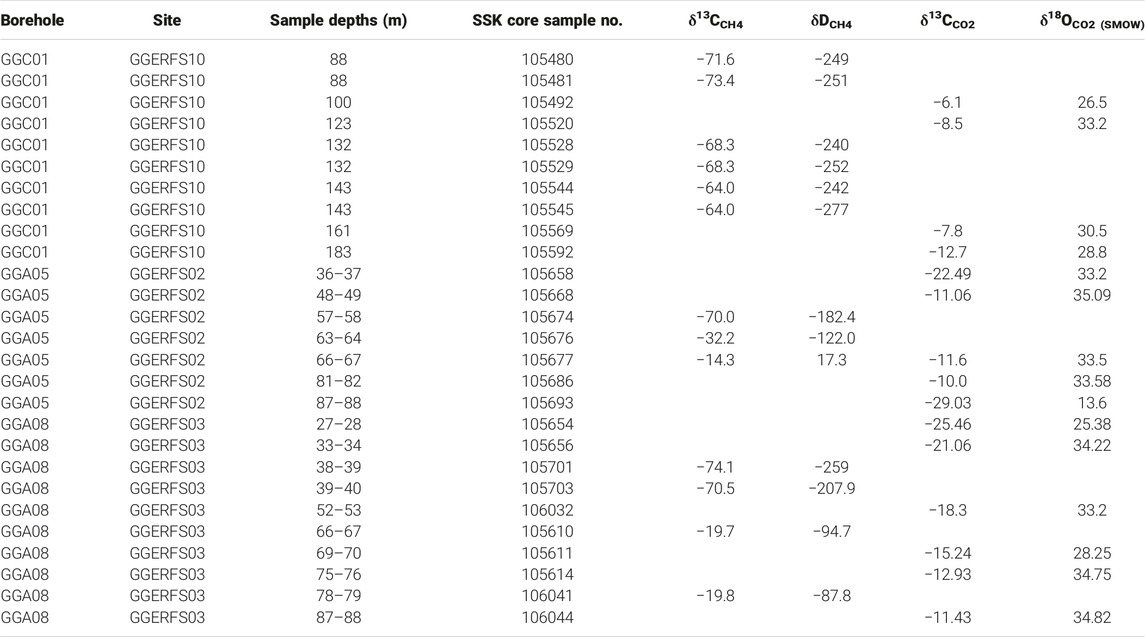
TABLE 1. Measured C-H-O isotope values of CH4 and CO2 collected from GGERF site from boreholes GGC01, GGA05, and GGA08.
Carbon isotope compositions for CO2 range from −12.7‰ to −6.1‰ for GGC01; −29‰ to −10‰ for GGA05; and −25.5‰ to −11.4‰ for GGA08. Such values align with previously reported values of coal bed globally (δ13CCO2 = −27‰ to +19‰) (Rice, 1993). The carbon isotope values of Dissolved Inorganic Carbon (δ13CDIC) of produced waters were obtained during pumping tests conducted by the BGS for the shallow mine monitoring boreholes. Analysis of these samples found that δ13CDIC in the groundwaters range from −12.8‰ to −7.1‰, with an average value of −10.9‰ (Palumbo-Roe et al., 2021). For the Midland Valley of Scotland, these results fall within the upper range of values previously recorded for coal measures (Palumbo-Roe et al., 2021).
Discussion
Subsurface CH4 Sources at the Glasgow Observatory
Figure 6 shows the genetic δ13CCH4 and δDCH4 diagram by Milkov and Etiope (2018) for GGC01, GGA05, and GGA08 highlighting evidence for two distinct CH4 signatures. The majority of samples show evidence for the production of CH4 by carbonate reduction, with the indication of the addition of minor amounts of CH4 produced through methyl-type fermentation. Through plotting isotopic values of CH4 and CO2 from the same stratigraphic unit (Figure 7), it is evident that the majority of samples exhibit a greater 13C enrichment, with an isotopic fractionation >55% for 13CCH4 relative to 13CCO2. This is indicative of CH4 production primarily by carbonate reduction (Whiticar, 1999). The 13CCH4 and 2HCH4 enriched samples appear to plot in the thermogenic CH4 origin field of Figure 6. However, previous studies have consistently shown that during CH4 oxidation, 12C is preferentially removed resulting in a marked decrease in isotopic fractionation between CH4 and CO2, and during advanced stages, this fractionation can range between 5%–25% (Barker and Fritz, 1981; Whiticar, 1999). Figure 7 highlights the difference Δ13CCO2-CH4 is close to 5‰, and follows the evolution pathway for CH4 oxidation. Hence, this data implies that bacterial CH4 is originally generated from high organic content sedimentary units and coals under anoxic conditions primarily via the carbonate reduction pathway (Krüger et al., 2008; Guo et al., 2012; Gründger et al., 2015). Subsequently, at 63–79 m depth at the GGERF site, the oxidative consumption of bacterial coal bed CH4 occurs resulting in a distinctly enriched 13C and 2H CH4 signature.
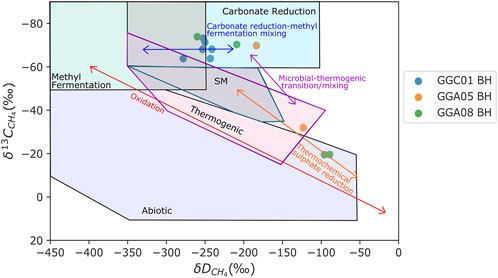
FIGURE 6. Plot of δDCH4 and δ13CCH4 stable isotopic analyses of CH4 gas exsolved from core and cutting samples from GGC01, GGA05, and GGA08 boreholes. (Secondary CH4 (SM) boundary indicated in green and thermogenic CH4 boundary indicated in purple). Processes that affect the isotopic and molecular composition are highlighted (oxidation and thermochemical sulphate reduction). Mixing of microbial gases produced through carbonate reduction and methyl fermentation is indicated by the blue mixing arrow, with mixing of thermogenic and microbial methane indicated by the purple mixing arrow. The majority of samples plot within the biogenic CH4 zone, with a potential mixing of both carbonate reduction and methyl type fermentation sources. Enriched samples plotting outside of biogenic origin fields are a result of CH4 oxidation. The classification areas of biogenic and thermogenic CH4 sources are adapted from Whiticar (1999), and the plot is adapted from Milkov and Etiope (2018).
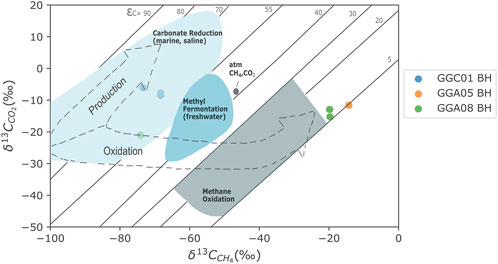
FIGURE 7. Isotope combination plot of δ13CCH4 and δ13CCO2 data from GGC01, GGA05, and GGA08 boreholes; with isotope fractionation lines and partitioning trajectories as a result of CH4 formation and oxidation processes. The majority of samples exhibit greater 12C enrichment with an isotopic fractionation indicative of CH4 production by carbonate reduction. The three enriched samples that plot around 5% isotope fractionation indicate CH4 oxidation, as 12C is preferentially removed resulting in a decrease in isotopic fractionation between 13CCH4 relative to 13CCO2. Isotope plot adapted from Whiticar (1999).
CO2 Signatures
Sources of CO2 gas within coal beds are dependent on the burial and uplift history of the stratigraphic units, and may also contain CO2 contributions from other sources such as dissolved atmospheric and soil gas, magmatic or mantle degassing, microbial degradation of organic substrates, and the thermal maturation of kerogen (Dai et al., 1996; Golding et al., 2013). In relation to interpreting δ13CCO2 and α13CCO2-CH4 values, there are a number of non-methanogenic processes that can affect gas signatures and therefore shift α13CCO2-CH4 from the “true” methanogenic fractionation value (Flores et al., 2008; Golding et al., 2013; Baublys et al., 2015; Vinson et al., 2017; Chen et al., 2023). Such processes include the mixing of biogenic and thermogenic gases, methane oxidation resulting in the conversion of CH4 to CO2, bacterial processes which produce CO2 such as sulfate reduction, and interaction with formation waters resulting in gas losses (Whiticar et al., 1986; Golding et al., 2013; Vinson et al., 2017). The mixing of biogenic and thermogenic gases can lower the α13CCO2-CH4 value, as thermogenic gas typically has a more enriched δ13CCH4 and depleted δ13CCO2 signature than biogenic gas (Whiticar et al., 1986; Vinson et al., 2017). Methane oxidation affects the α13CCO2-CH4 values as the residual un-oxidated methane has a more enriched δ13CCH4 signature and therefore lowers the apparent α13CCO2-CH4 value (Whiticar, 1999; Vinson et al., 2017; Chen et al., 2023). Bacterial processes such as sulfate reduction can consume CH4 and produce CO2, with little fractionation on the carbon values, resulting in the lowering of the α13CCO2-CH4 value (Vinson et al., 2017). Finally, CH4 and CO2 can be lost through dissolution and advection as groundwater flows through the coal bed formation. Therefore, a semi-open system where CH4 and CO2 are not fully retained results in the α13CCO2-CH4 value being affected (Golding et al., 2013; Vinson et al., 2017).
Microbial coal bed gases tend to have carbon and hydrogen fractionation factors [α13CCO2-CH4= (1,000 + δ13CCO2)/(1,000 + δ13CCH4)] close to expected α13CCO2-CH4 values for the carbonate reduction pathway (1.06–1.09) (Golding et al., 2013; Vinson et al., 2017; Chen et al., 2023). 13CCO2 and 13CCH4 values from close stratigraphic horizons, highlighted in Figure 7, indicate a consistent α13CCO2-CH4 value of 1.06 for all GGC01 samples, and for some shallow samples from GGA05 and GGA08, indicating a characteristic CO2 reduction pathway for methanogenesis. However, several GGA05 and GGA08 samples from 66 to 79 m depth have much lower α13CCO2-CH4 values (1.003–1.007), evidencing the potential for non-methanogenic processes altering the “true” methanogenic fractionation factor. The dissolution of microbial CO2 results in enriched δ13CDIC values of 8‰ relative to the gas phase CO2 (Clark and Fritz, 1997). With pumping test data (Palumbo-Roe et al., 2021) establishing measured δ13CDIC values of −12.8‰ to −7.1‰ within groundwater contained in the mineworkings, a general enrichment of 8‰ can be observed between δ13CDIC and δ13CCO2 values (For example: superficial deposits δ13CDIC = −12.8‰ to −10.9‰ and δ13CCO2 = −25.5‰ to −21‰; Glasgow Upper δ13CDIC = −11.2‰ to −10.9‰ and δ13CCO2 = −18.3‰; Glasgow Main δ13CDIC=−10.8‰ and δ13CCO2 = −19.8‰). The additional enrichment of δ13CDIC observed in the samples may be explained by interaction with carbonates via precipitation and dissolution reactions, the source of which may potentially derive from sulphuric acid produced through pyrite oxidation within the former coal mine workings (Palumbo-Roe et al., 2021). As such, the varying δ13CCO2 signatures within the site highlight a potential for a combination of non-methanogenic processes such as methane oxidation and dissolution occurring, highlighting the complications of using isotopic identification techniques.
Stable Isotope Profiles With Depth
The stable isotope profiles with depth are plotted for CH4 and CO2 in Figure 8, illustrating that there is no clear correlation of the CH4 stable isotope ratios with depth. There is a consistent biogenic δ13CCH4 signature of −75‰ to −64‰, with a distinct zone of markedly heavier CH4 occurring between a depth of 63 and 79 m, corresponding to enriched δ13CCH4 values of −32.2‰ and −14.3‰. At Borehole GGA05, the heavily oxidised signatures occur within 5–9 m above the collapsed Glasgow Ell coal mine workings, in both clay and silt sedimentary units that contain thin coal seams and have high organic content. The enriched 13CCH4 signatures for GGA08 are found in clay, silt, and sand sedimentary units, with moderate organic content within 3–9 m of the same Glasgow Ell workings. In both GGA05 and GGA08 boreholes, enriched 13CCH4 and 2HCH4 signatures are observed at 66–67 m depths, hence it is unlikely this enriched signature is the result of air ingress into isojars during sample storage.
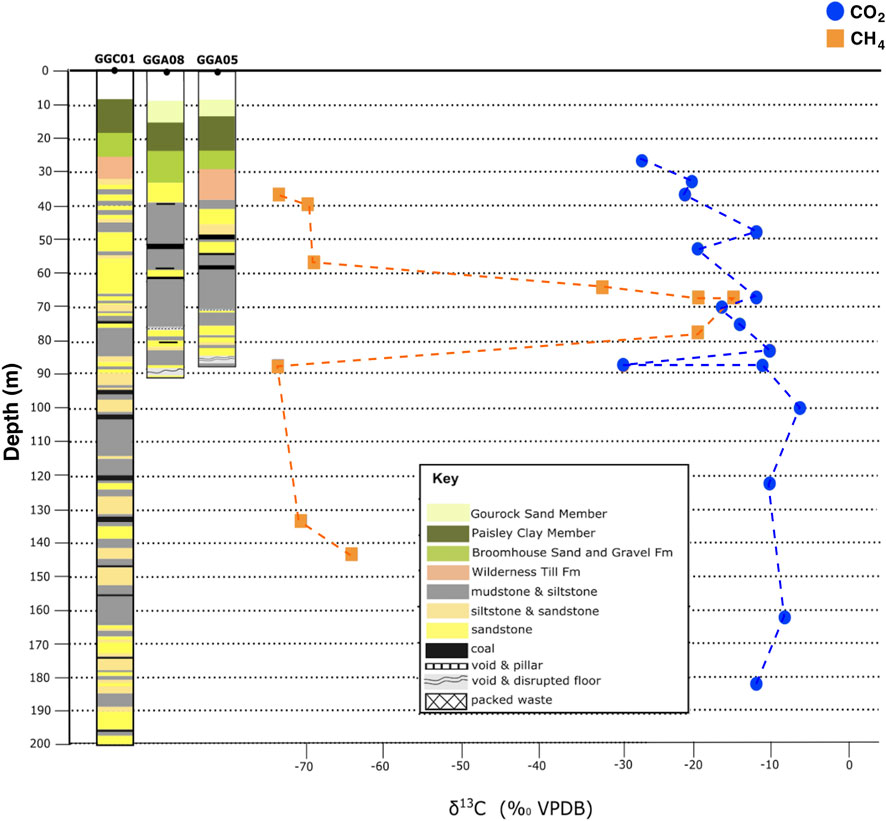
FIGURE 8. Isotopic depth plots of CH4 and CO2 δ13C values from GGC01, GGA05, and GGA08 boreholes; with the corresponding stratigraphy. CH4 stable isotopes have no clear correlation with depth, with a consistent biogenic signature present, and a distinct zone of enriched CH4 in the area surrounding the Glasgow Ell mine workings. CO2 gas exhibits a consistent depleted 13CCO2 signature with shallower depth, highlighting the increasing influence of shallow groundwater within the subsurface.
A consistent signature was observed in the CO2 from these samples, with CO2 gas signatures showing a progressive depletion in 13CCO2 at shallower depths [from ∼−10‰ at 180 m depth to −23‰ in the shallowest sample (36 m depth)] (Figure 8). Values recorded from the superficial deposits are the most depleted in 13CCO2, with the unmined bedrock samples from GGC01 being the most enriched in 13C, with the exception of a distinct depleted CO2 sample (−29‰) occurring at ∼90 m depth, in the area of the Glasgow Main mine-workings.
Lessons for the Monitoring of Minewater Geothermal Sites
In establishing the environmental baseline of the site, ground gas baseline surveys were also undertaken at the Glasgow Observatory, in order to determine if potential mine gases or gas originating from overlying made ground could be detected in the near surface environment (Monaghan et al., 2022a). Through these surveys, CO2 and CH4 flux at the soil-atmosphere interface, ground gas concentrations of CO2, CH4, H2, H2S, O2, a proxy for N2, and a limited number of carbon stable isotope samples were measured (see Monaghan et al., 2022b for full sampling methodology).
Ground gas CH4 concentrations were comparable to atmospheric gas (<3 ppm by volume) and CH4 flux was typically below detection limits (Monaghan et al., 2022a), which corresponds well to our measured CH4 concentration data, with the majority of samples having CH4 levels below detection limits, and the highest CH4 concentration levels recorded in the areas of unmined coal seams, or the Glasgow Ell mine workings. CO2 flux measured above the site was consistent with uncontaminated rural (Ward et al., 2019) and other UK sites previously surveyed. However, there were instances of moderate ground gas concentrations (10%–20% by volume) in isolated points across surveys (Monaghan et al., 2022b). From limited carbon stable isotope ratios, δ13C values typically range from −23.59‰ to −26.31‰ and compare well to our shallowest GGA05 and GGA08 samples, as δ13CCO2 values get progressively lower to a value of −23.0‰, as soil gas CO2 has an increasing influence through shallow groundwaters. This also highlights gas concentrations and signatures are highly variable and closely linked to stratigraphic horizon in the shallow subsurface, as it is evident that mine gas signatures from the workings does not impact ground gas (Monaghan et al., 2022a). From stoichiometric CO2: O2 relationships, ground gas appears to be a mixture of natural origin of photosynthetic production, and of microbial oxidation of CH4 to CO2 (Monaghan et al., 2022b).
The comparison of ground gas data with core and cutting gas measurements is critical for the monitoring of geothermal and other geoenergy activities, as it allows for the sensitive measuring and tracking of key hazardous gases that may arise from subsurface use (Monaghan et al., 2022a). Our results show that the CO2 contained in the subsurface below 100 m depth is geochemically distinct from that of the shallow subsurface (0–90 m depth), meaning that an increase of CO2 levels at the near-surface originating from deeper mine workings below 100 m from any potential perturbation of the system may be detectable using δ13C measurements. However, further work is required to ascertain the detection limit, and if gas migration processes would significantly change the δ13C signature of the migrating CO2.
Conclusion
We identify the presence of both CH4 and CO2 in the gases exsolved from core and cutting samples taken from boreholes GGC01, GGA05, and GGA08 at the Glasgow Observatory site. Our results show that there is no correlation between gas concentration and depth, as both CH4 and CO2 gas concentration values are highly variable and are closely linked to individual stratigraphic horizons. We find evidence that CH4 present in the site’s Carboniferous coal measures is of biogenic origin, produced primarily through the carbonate reduction pathway, with a potential mixing of CH4 from methyl-type fermentation. Enriched 13C and 2H CH4 signatures are found within 63–79 m depth in GGA05 and GGA08 boreholes, and provide evidence of CH4 oxidation in proximity to the Glasgow Ell coal mine workings. CO2 gas is more abundant throughout the succession in all three boreholes and has an enriched 13CCO2 signature relative to the CH4 present. The observed CO2 gas signature becomes progressively depleted in 13CCO2 at shallower depths above 90 m, with the trend being attributed to the increasing influence of groundwater containing a mixture of dissolved marine carbonate minerals and soil gas CO2 at shallower depths. Comparing our results to determined ground gas signatures, there is no evidence of ground gas currently being impacted by gas migration from the Glasgow Observatory mine workings.
The findings presented here provide an insight into the variability of mine derived gases, and highlight the presence of distinct gas signatures that are linked to stratigraphic horizon. The gas baseline signature of the shallow subsurface of the Glasgow Observatory can be integrated into larger environmental datasets (Monaghan et al., 2022b) in order to generate a “time zero” records of the site, which are key in informing fit-for-purpose monitoring operations and developing efficient geothermal infrastructures. By characterising the shallow subsurface through depth-dependent isotopic gas fingerprints in the mined succession and comparing to distinctive ground gas isotopic compositions; there is potential to use such signatures to evaluate any potential change in the shallow subsurface environment once pumping, heat abstraction, and re-injection commence.
Data Availability Statement
The datasets presented in this study can be found in online repositories. The names of the repository/repositories and accession number(s) can be found in the article/Supplementary Material.
Author Contributions
RC, GJ, and SG: conceptualisation, investigation, writing, and visualisation; AB conceptualisation, investigation and reviewing writing. RC: funding acquisition. All authors have read and agreed to the published version of the manuscript.
Funding
The funding came from the NERC UK Geoenergy Observatories Research Internship programme (2019–2020) (Grant Reference Number Nu000072), whose support is gratefully acknowledged. Samples and data are derived from the UK Geoenergy Observatories Programme funded by the UKRI Natural Environment Research Council and delivered by the British Geological Survey (UKGEOS Core Loan Numbers 267644 and 272269).
Conflict of Interest
The authors declare that the research was conducted in the absence of any commercial or financial relationships that could be construed as a potential conflict of interest.
Publisher’s Note
All claims expressed in this article are solely those of the authors and do not necessarily represent those of their affiliated organizations, or those of the publisher, the editors and the reviewers. Any product that may be evaluated in this article, or claim that may be made by its manufacturer, is not guaranteed or endorsed by the publisher.
Acknowledgments
The authors are grateful to the British Geological Survey (BGS), with special thanks to Alison Monaghan and others involved in establishing the UKGEOS Glasgow project, and for collection of samples on site. Thanks to David Manning and Karen Scrivens at Newcastle for facilitating the internship and associated funding. Thanks also to Terry Donnelly for stable isotope analysis at the Scottish Universities Environmental Research Centre (SUERC). GJ acknowledges funding from the Faculty of Engineering at the University of Strathclyde.
Supplementary Material
The Supplementary Material for this article can be found online at: https://www.escubed.org/articles/10.3389/esss.2023.10073/full#supplementary-material
References
Adams, C., Monaghan, A., and Gluyas, J. (2019). Mining for Heat. Geoscientist 29 (4), 10–15. doi:10.1144/geosci2019-021
Barker, J. F., and Fritz, P. (1981). Carbon Isotope Fractionation during Microbial Methane Oxidation. Nature 293 (5830), 289–291. doi:10.1038/293289a0
Baublys, K. A., Hamilton, S., Golding, S., Vink, S., and Esterle, J. (2015). Microbial Controls on the Origin and Evolution of Coal Seam Gases and Production Waters of the Walloon Subgroup; Surat Basin, Australia. Int. J. Coal Geol. 147-148, 85–104. doi:10.1016/j.coal.2015.06.007
British Geological Survey (2020a). UKGEOS Glasgow GGA05 Borehole Information Pack. Nottingham, United Kingdom: British Geological Survey. Dataset.
British Geological Survey (2020b). UKGEOS Glasgow GGA08 Borehole Information Pack. Nottingham, United Kingdom: British Geological Survey. Dataset.
Cameron, I. B., and Stephenson, D. (1985). British Regional Geology: The Midland Valley of Scotland (No. 5). London, United Kingdom; Hmso Books.
Chen, X., Wang, Y., Tao, M., Zhou, Z., He, Z., and Song, K. (2023). Tracing the Origin and Formation Mechanisms of Coalbed Gas from the Fuxin Basin in China Using Geochemical and Isotopic Signatures of the Gas and Coproduced Water. Int. J. Coal Geol. 267, 104185. doi:10.1016/j.coal.2023.104185
Cl:Aire, (2021). Good Practice for Risk Assessment for Coal Mine Gas Emissions. Buckinghamshire, United Kingdom: CL:AIRE.
Clark, I. D., and Fritz, P. (1997). Environmental Isotopes in Hydrogeology. Florida, United States: CRC Press.
Coplen, T. B. (1995). Reporting of Stable Carbon, Hydrogen, and Oxygen Isotopic Abundances. Reference Intercomp. Mater. stable isotopes light Elem. 825, 31–34. doi:10.1351/pac199466020273
Craig, H. (1957). Isotopic Standards for Carbon and Oxygen and Correction Factors for Mass-Spectrometric Analysis of Carbon Dioxide. Geochimica cosmochimica acta 12 (1-2), 133–149. doi:10.1016/0016-7037(57)90024-8
Dai, J. X., Song, Y., and DaiSenWang, C. D. R. (1996). Geochemistry and Accumulation of Carbon Dioxide Gases in China. Am. Assoc. Pet. Geol. Bull. 80, 1615–1626. doi:10.1306/64EDA0D2-1724-11D7-8645000102C1865D
Dochartaigh, B. Ó., Bonsor, H., and Bricker, S. (2019). Improving Understanding of Shallow Urban Groundwater: the Quaternary Groundwater System in Glasgow, UK. Earth Environ. Sci. Trans. R. Soc. Edinb. 108 (2-3), 155–172. doi:10.1017/S1755691018000385
Donnelly, T., Waldron, S., Tait, A., Dougans, J., and Bearhop, S. (2001). Hydrogen Isotope Analysis of Natural Abundance and Deuterium-enriched Waters by Reduction over Chromium On-line to a Dynamic Dual Inlet Isotope-ratio Mass Spectrometer. Rapid Commun. Mass Spectrom. 15 (15), 1297–1303. doi:10.1002/rcm.361
Dunbar, E., Cook, G. T., Naysmith, P., Tripney, B. G., and Xu, S. (2016). AMS 14 C Dating at the Scottish Universities Environmental Research Centre (SUERC) Radiocarbon Dating Laboratory. Radiocarbon 58 (1), 9–23. doi:10.1017/rdc.2015.2
Flores, R. M., Rice, C. A., Stricker, G. D., Warden, A., and Ellis, M. S. (2008). Methanogenic Pathways of Coal-Bed Gas in the Powder River Basin, United States: the Geologic Factor. Int. J. coal Geol. 76 (1-2), 52–75. doi:10.1016/j.coal.2008.02.005
Golding, S. D., Boreham, C. J., and Esterle, J. S. (2013). Stable Isotope Geochemistry of Coal Bed and Shale Gas and Related Production Waters: A Review. Int. J. Coal Geol. 120, 24–40. doi:10.1016/j.coal.2013.09.001
Gonfiantini, R. (1984). Stable Isotope Reference Samples for Geochemical and Hydrological Investigations. Int. J. Appl. Radiat. Isot.;(United Kingdom) 35 (5), 426. doi:10.1016/0020-708x(84)90059-0
Gründger, F., Jiménez, N., Thielemann, T., Straaten, N., Lüders, T., Richnow, H. H., et al. (2015). Microbial Methane Formation in Deep Aquifers of a Coal-Bearing Sedimentary Basin, Germany. Front. Microbiol. 6, 200. doi:10.3389/fmicb.2015.00200
Guo, H., Liu, R., Yu, Z., Zhang, H., Yun, J., Li, Y., et al. (2012). Pyrosequencing Reveals the Dominance of Methylotrophic Methanogenesis in a Coal Bed Methane Reservoir Associated with Eastern Ordos Basin in China. Int. J. coal Geol. 93, 56–61. doi:10.1016/j.coal.2012.01.014
Györe, D., McKavney, R., Gilfillan, S. M., and Stuart, F. M. (2018). Fingerprinting Coal-Derived Gases from the UK. Chem. Geol. 480, 75–85. doi:10.1016/j.chemgeo.2017.09.016
Hall, J., Glendinning, S., and Younger, P. (2005). “Is Mine Water a Source Of Hazardous Gas?,” in 9th International Mine Water Congress, Asturias, Spain, September 2005, 5–7.
Jackson, R. E., Gorody, A. W., Mayer, B., Roy, J. W., Ryan, M. C., and Van Stempvoort, D. R. (2013). Groundwater Protection and Unconventional Gas Extraction: The Critical Need for Field-based Hydrogeological Research. Groundwater 51 (4), 488–510. doi:10.1111/gwat.12074
Krüger, M., Beckmann, S., Engelen, B., Thielemann, T., Cramer, B., Schippers, A., et al. (2008). Microbial Methane Formation from Hard Coal and Timber in an Abandoned Coal Mine. Geomicrobiol. J. 25 (6), 315–321. doi:10.1080/01490450802258402
Kusakabe, M. (2005). A Closed Pentane Trap for Separation of SO2 from CO2 for Precise δ18O and & δ34S Measurements. Geochem. J. 39 (3), 285–287. doi:10.2343/geochemj.39.285
LeDoux, S. T., Szynkiewicz, A., Faiia, A. M., Mayes, M. A., McKinney, M. L., and Dean, W. G. (2016). Chemical and Isotope Compositions of Shallow Groundwater in Areas Impacted by Hydraulic Fracturing and Surface Mining in the Central Appalachian Basin, Eastern United States. Appl. Geochem. 71, 73–85. doi:10.1016/j.apgeochem.2016.05.007
Milkov, A. V., and Etiope, G. (2018). Revised Genetic Diagrams for Natural Gases Based on a Global Dataset Of> 20,000 Samples. Org. Geochem. 125, 109–120. doi:10.1016/j.orggeochem.2018.09.002
Molofsky, L. J., Connor, J. A., Wylie, A. S., Wagner, T., and Farhat, S. K. (2013). Evaluation of Methane Sources in Groundwater in Northeastern Pennsylvania. Groundwater 51 (3), 333–349. doi:10.1111/gwat.12056
Monaghan, A. A., Damaschke, M., Starcher, V., Fellgett, M. W., Kingdon, A., Kearsey, T., et al. (2021). UKGEOS Glasgow GGC01 Final Borehole Information Pack. National Geoscience Data Centre. NERC EDS (Dataset).
Monaghan, A. A., Bateson, L., Boyce, A. J., Burnside, N. M., Chambers, R., De Rezende, J. R., et al. (2022a). Time Zero for Net Zero: a Coal Mine Baseline for Decarbonising Heat. Earth Sci. Syst. Soc. 2, 9. doi:10.3389/esss.2022.10054
Monaghan, A. A., Starcher, V., Barron, H. F., Shorter, K., Walker-Verkuil, K., Elsome, J., et al. (2022b). Drilling into Mines for Heat: Geological Synthesis of the UK Geoenergy Observatory in Glasgow and Implications for Mine Water Heat Resources. Q. J. Eng. Geol. Hydrogeology 55 (1), 1–19. doi:10.1144/qjegh2021-033
Monaghan, A. A., Starcher, V., O Dochartaigh, B., Shorter, K., and Burkin, J. (2019). UK Geoenergy Observatories: Glasgow Geothermal Energy Research Field Site: Science Infrastructure Version 2. Nottingham, United Kingdom: British Geological Survey.
NERC, University of Strathclyde and BGS (2019). Record of Proceedings UK Geoenergy Observatories Glasgow Geothermal Energy Innovation Workshop. Available from: https://cms.ukgeos.ac.uk/event/assets/innovation_event_030619_ final.pdf (Accessed 20 December, 2022).
Osborn, S. G., Vengosh, A., Warner, N. R., and Jackson, R. B. (2011). Methane Contamination of Drinking Water Accompanying Gas-Well Drilling and Hydraulic Fracturing. Proc. Natl. Acad. Sci. 108 (20), 8172–8176. doi:10.1073/pnas.1100682108
Palumbo-Roe, B., Shorter, K. M., Fordyce, F. M., Walker-Verkuil, K., O Dochartaigh, B. E., Gooddy, D. C., et al. (2021). UK Geoenergy Observatories: Glasgow Borehole Test Pumping-Groundwater Chemistry. Nottingham, United Kingdom: British Geological Survey
Ramsay, C., McRae, C., Ryan, E., McCallum, A., Wellington, L., Lauder, L., et al. (2017). Carbon Dioxide Ingress into Residential Houses at Gorebridge in Midlothian, Scotland, United Kingdom. Eur. J. Public Health 27, 108–109. doi:10.1093/eurpub/ckx187.278
Rice, D. D. (1993). Composition and Origins of Coalbed Gas. Hydrocarbons coal AAPG Stud. Geol. 38 (1), 159–184. doi:10.1306/St38577C7
Schoell, M. (1980). The Hydrogen and Carbon Isotopic Composition of Methane from Natural Gases of Various Origins. Geochimica Cosmochimica Acta 44 (5), 649–661. doi:10.1016/0016-7037(80)90155-6
Stephenson, M. H., Ringrose, P., Geiger, S., Bridden, M., and Schofield, D. (2019). Geoscience and Decarbonization: Current Status and Future Directions. Pet. Geosci. 25 (4), 501–508. doi:10.1144/petgeo2019-084
Stolper, D. A., Lawson, M., Formolo, M. J., Davis, C. L., Douglas, P. M., and Eiler, J. M. (2018). The Utility of Methane Clumped Isotopes to Constrain the Origins of Methane in Natural Gas Accumulations. Geol. Soc. Lond. Spec. Publ. 468 (1), 23–52. doi:10.1144/sp468.3
Strąpoć, D., Mastalerz, M., Dawson, K., Macalady, J., Callaghan, A. V., Wawrik, B., et al. (2011). Biogeochemistry of Microbial Coal-Bed Methane. Annu. Rev. Earth Planet. Sci. 39, 617–656. doi:10.1146/annurev-earth-040610-133343
Stuart, M. E. (2012). “Potential Groundwater Impact from Exploitation of Shale Gas in the UK,”. Report No: OR/12/001 (Nottingham, United Kingdom: British Geological Survey Open Report), 33.
Vinson, D. S., Blair, N. E., Martini, A. M., Larter, S., Orem, W. H., and McIntosh, J. C. (2017). Microbial Methane from In Situ Biodegradation of Coal and Shale: A Review and Reevaluation of Hydrogen and Carbon Isotope Signatures. Chem. Geol. 453, 128–145. doi:10.1016/j.chemgeo.2017.01.027
Ward, R. S., Smedley, P. L., Allen, G., Baptie, B. J., Barkwith, A. K. A. P., Bateson, L., et al. (2019). “Environmental Monitoring - Phase 4 Final Report (April 2018 - March 2019),”. Report No: OR/19/044 (Nottingham, United Kingdom: British Geological Survey), 225. Open Report.
Whiticar, M. J. (1999). Carbon and Hydrogen Isotope Systematics of Bacterial Formation and Oxidation of Methane. Chem. Geol. 161 (1-3), 291–314. doi:10.1016/s0009-2541(99)00092-3
Keywords: geochemistry, geothermal, mine water, environmental monitoring, geoenergy
Citation: Chambers RM, Johnson G, Boyce AJ and Gilfillan SMV (2023) Constraining the Geochemical Fingerprints of Gases from the UK Carboniferous Coal Measures at the Glasgow Geoenergy Observatories Field Site, Scotland. Earth Sci. Syst. Soc. 3:10073. doi: 10.3389/esss.2023.10073
Received: 22 December 2022; Accepted: 08 May 2023;
Published: 22 May 2023.
Edited by:
Jack Longman, University of Oldenburg, GermanyReviewed by:
Xiaoqiang Li, The University of Texas at Austin, United StatesYunpeng Wang, Guangzhou Institute of Geochemistry, Chinese Academy of Sciences (CAS), China
Qiong Wang, China University of Geosciences, China
Julie K. Pearce, The University of Queensland, Australia
Copyright © 2023 Chambers, Johnson, Boyce and Gilfillan. This is an open-access article distributed under the terms of the Creative Commons Attribution License (CC BY). The use, distribution or reproduction in other forums is permitted, provided the original author(s) and the copyright owner(s) are credited and that the original publication in this journal is cited, in accordance with accepted academic practice. No use, distribution or reproduction is permitted which does not comply with these terms.
*Correspondence: Rebecca M. Chambers, rebecca.chambers@ed.ac.uk