- 1Department of Civil Engineering, Monash University, Melbourne, VIC, Australia
- 2Hydrogen Initiatives, Department of Industry, Science, Energy and Resources, Canberra, ACT, Australia
- 3Geoscience Australia, Canberra, ACT, Australia
Australia has ambitions to become a major global hydrogen producer by 2030. The establishment of Australia’s and the world’s hydrogen economy, however, will depend upon the availability of affordable and reliable hydrogen storage. Geological hydrogen storage is a practical solution for large scale storage requirements ensuring hydrogen supply can always meet demand, and excess renewable electricity can be stored for later use, improving electricity network reliability. Hosting thick, underground halite (salt) deposits and an abundance of onshore depleted gas fields, Australia is well placed to take advantage of geological hydrogen storage options to support its ambition of building a global hydrogen hub export industry. Using the Bluecap modelling software, we identify regions in Australia that are potentially profitable for large scale hydrogen production and storage. We use the results of this work to suggest high-potential regions for hydrogen development, supporting policymaker and investor decisions on the locations of new infrastructure and hydrogen projects in Australia.
Introduction
Hydrogen is a clean-burning fuel and an essential reducing agent with the potential to decarbonise hard-to-abate industrial sectors such as steel and aluminium production, long-haul transport, and industrial heat (IEA, 2019). Governments around the world are increasingly relying upon the establishment of a global hydrogen industry to meet their greenhouse gas (GHG) emissions reduction targets, including many of the countries and jurisdictions that have pledged net-zero emissions by the middle of the century (Bouckaert et al., 2021). A detailed review of many of these policies can be found in the 2022 State of Hydrogen Report, released by the Australian government (DCCEEW, 2022). Australia has ambitions to become a major global hydrogen producer by 2030 (DISER, 2021) and, if successful, would be in strong position to meet its currently pledged climate commitments while significantly contributing to the international race to net-zero emissions by 2050 (COAG Energy Council, 2019).
Endowed with world-class renewable energy resources, a steady supply of natural gas, suitable sites for carbon capture and storage (CCS) and a track record of building large-scale energy industries, Australia is well placed to establish a sustainable domestic and export hydrogen industry (Bruce et al., 2018a; COAG Energy Council, 2019). A key component of Australia’s National Hydrogen Strategy is the development of ‘hydrogen hubs’—regions where large-scale hydrogen demand is aggregated through the co-location of hydrogen users, producers and exporters (COAG Energy Council, 2019; DISER, 2021). Hubs can facilitate the scaling up of hydrogen deployment as the co-location of hydrogen supply and demand reduces upfront investment in transmission and distribution infrastructure, reducing overall hydrogen pathway costs (IEA, 2019). However, the establishment of a hydrogen industry in Australia and globally will depend upon the availability of affordable and reliable large-scale, hydrogen storage solutions (BNEF, 2020).
Geoscience Australia and Monash University have developed the Hydrogen Economic Fairways Tool (HEFT) to assess regions of high potential for the development of new large-scale hydrogen projects (Walsh et al., 2021).1 The model forms part of the Bluecap software suite—a set of tools for evaluating the regional economic potential for new resource project developments (Walsh et al., 2020).2 HEFT accounts for the costs associated with hydrogen production, the quality of the input energy resources, as well as the availability of local infrastructure to support the project and the distance to the final point of sale.
This paper describes how the Bluecap modelling software has been extended to identify regions in Australia that are potentially profitable for large-scale hydrogen production and storage. The new model considers the potential for storage of hydrogen from renewable and non-renewable sources in salt caverns and depleted gas fields. It accounts for the proximity to the storage locations, the relative costs of storage and the availability of local infrastructure. We use the results of this work to suggest high-priority regions for hydrogen development, supporting policymaker and investor decisions on the locations of new infrastructure and hydrogen projects in Australia.
Geological Hydrogen Storage
One of the major challenges in the global race to net-zero emissions is the need for large-scale energy storage to stabilise and improve reliability of intermittent renewable energy supply (Bouckaert et al., 2021; CSIRO, 2021; IRENA, 2021). Hydrogen can contribute significantly to overcoming this challenge as it can be stored in large quantities and over long time periods (BNEF, 2020). For example, excess renewable electricity can be converted into hydrogen via electrolysis—which is then stored, and converted back into electricity as required (COAG Energy Council, 2019; CSIRO, 2021). Stored hydrogen can also be used to balance seasonal fluctuations in the natural gas network (CSIRO, 2021; IRENA, 2021) and could, in the future, help hydrogen exporters meet their shipping schedules (CSIRO, 2021).
The most common hydrogen storage method used today is compression via pressurisation in steel or carbon composite cylinders for small scale applications (Makridis, 2016; IEA, 2019). Pipeline storage, solid state hydrogen storage, and above ground tanks are also suitable for small scale and short term storage requirements (Andersson and Grönkvist, 2019; IEA, 2019). The capacities of these storage methods range from a few kilograms to several tonnes per kilometer in the case of pipeline storage (Kruck et al., 2013). Small capacity storage methods, however, are costly when used on large scales (i.e., 100s of tonnes) due to the relatively low volumetric energy-density of hydrogen compared to other fuels (Bruce et al., 2018a). Underground geological storage options, therefore, offer a practical and cost effective alternative (Andersson and Grönkvist, 2019) and are considered the best option for large-scale and long-term storage of hydrogen (Kruck et al., 2013; Simon et al., 2015; IEA, 2019).
Geological hydrogen storage options include salt caverns, depleted gas fields, rock caverns and aquifers (Tarkowski, 2019). Salt cavern storage and depleted gas fields are generally considered to be the most advanced of these four options: Salt cavern storage is currently in use for long-term hydrogen storage (Hevin, 2019), and while only a few depleted gas fields have been trialed for hydrogen storage, they are widely used for natural gas storage (Craig et al., 2022). Rock caverns and saline aquifers have also been suggested as options for hydrogen storage. However, these options face additional challenges. Rock caverns must be lined (e.g., with steel and cement) to prevent leakage. This increases the initial cost of investment relative to salt caverns, and means that rock caverns will not self-seal after tectonic activity (Khaledi et al., 2016; Ennis-King et al., 2021). Aquifer storage has many similarities with storage in gas reservoirs, with the additional complication that there is not guarantee of a trapping mechanism (Craig et al., 2022). In addition, the reservoir properties of many saline aquifers will be less well known than those in depleted gas reservoirs, requiring additional development time. As such, although underground rock cavern and aquifer hydrogen storage may have future potential, these technologies are less well established (Muhammed et al., 2022) and are not considered in the assessment below.
As suitable sites for underground storage are dependent on the geographical distribution of the aforementioned storage options (IEA, 2019), geological storage will not be possible for all countries wanting to establish their own export-sized hydrogen supply chains (BNEF, 2020). Australia is well placed to take advantage of geological storage to support its ambition of becoming a major global hydrogen player by 2030, as the country hosts thick, underground halite (salt) deposits and an abundance of onshore depleted gas fields (Bruce et al., 2018a). The advantages and disadvantages of these two geological storage options are outlined in the following sections.
Salt Cavern Storage
Salt caverns are considered by many to be the best large scale storage option for hydrogen (Simon et al., 2015; Caglayan et al., 2020), if available, as they offer the lowest levelised cost per unit of hydrogen (Chen et al., 2023). Salt caverns are man-made structures, formed by drilling into a thick salt deposit, and circulating water to dissolve a section of the formation (Craig et al., 2022). Typical salt caverns sit deep underground (between 0.5–2 km), have a working capacity of 3,000–10,000 tonnes of hydrogen per cavern (BNEF, 2020), and are generally operated in a series of adjacent caverns (IEA, 2019). Caverns are a relatively mature storage technology, with thousands used to store oil, gas, hydrogen and other substances globally (BNEF, 2020). Long-running salt caverns used to store hydrogen include the Teesside caverns in the United Kingdom and the Clemens Dome, Spindletop and Moss Bluff caverns in the United States (Williams et al., 2022). The majority of internationally planned hydrogen storage facilities are also targeting salt caverns (Zivar et al., 2021).
The advantage of using caverns for hydrogen storage is they provide: significant economies of scale; high cycling rates and efficiencies (∼98%); low parasitic energy requirements; a low risk of hydrogen contamination and leakage due to the inert and impermeable nature of salt; low operational and land costs due to the small surface footprint of cavern infrastructure; and a low safety risk compared to other forms of hydrogen storage (Lord et al., 2014; Bunger et al., 2016; BNEF, 2020). Additionally, the pressures employed in cavern storage (45–275 bar; BNEF, 2020) enable high discharge rates making them attractive for industrial and power sector applications (IEA, 2019).
The main disadvantage is that the locations suitable for cavern storage are geographically limited due to their dependence on specific geology (Bruce et al., 2018a). Moreover, in places like Australia—where caverns are not yet used for commercial storage purposes—potentially expensive exploration campaigns are required (Bruce et al., 2018a). Caverns also require pipeline networks, which increase start-up costs, while the shrinking of a cavern may increase the cost of storage throughout a cavern’s lifetime (BNEF, 2020). From an environmental perspective, the cost of safely disposing of brines produced when creating a cavern must also be considered (Craig et al., 2022).
Depleted Gas Fields
Utilising depleted gas fields for natural gas storage is an economically viable technology with more than 500 facilities in operation globally (Judd and Pinchbeck, 2016). Use of depleted gas fields for hydrogen storage, however, is still at a low technological maturity and is not yet commercially viable. Only a few trial projects have been conducted globally (Ennis-King et al., 2021; Craig et al., 2022). The feasibility and cost of large-scale hydrogen storage in depleted gas fields, therefore, is yet to be proven (IEA, 2019). If this technology can be proven economically viable, it could serve as a suitable option for seasonal hydrogen storage, especially in locations with no access to salt caverns (IEA, 2019). With larger storage capacities (300–100,000 tonnes of hydrogen per field) (BNEF, 2020) and a wider distribution than caverns, depleted gas fields offer a suitable alternative to cavern storage (Bruce et al., 2018a).
A large benefit of storing hydrogen in depleted gas fields is that the geological properties of the storage reservoir are well understood as the locations have served as sites for gas production for several years (Craig et al., 2022). This reduces or eliminates the need for exploration and characterization (Bruce et al., 2018a). The maturity of the petroleum industry also means that much of the required infrastructure for operating a hydrogen storage facility (wells, pipelines etc.) is already in place, reducing capital costs compared to cavern storage (Ennis-King et al., 2021) (although it should be noted that some components may need to be lined or upgraded). Similar to caverns, depleted gas fields have low parasitic energy requirements and are considered a relatively safe technology (BNEF, 2020).
One of the main drawback of using depleted gas fields for storage is that they are geographically limited due to their dependence on geology (Ennis-King et al., 2021). Beyond location, the main challenge of using depleted gas fields for hydrogen storage is the risk of contamination. Hydrogen gas can mix with residual gases in the reservoir, reducing its purity (Amid et al., 2016; Ennis-King et al., 2021). Any such contaminants need to be removed before hydrogen can be used within a fuel cell, adding costs to the storage process (IEA, 2019). Other disadvantages of using depleted gas fields for hydrogen storage include (but are not limited to): hydrogen losses (approximately 2% per year; BNEF, 2020) through diffusion (Amid et al., 2016); the requirement of a high percentage (50%) of cushion gas (Kobos et al., 2011); and the slow cycling rate when compared with open caverns (Craig et al., 2022).
Locating Regions Favourable for Hydrogen Storage Development
Establishing a hydrogen industry in Australia and globally will depend upon the availability of affordable and reliable geological storage solutions (Geoscience Australia, 2021b). Careful planning is, therefore, required to ensure hydrogen supply networks (inclusive of geological storage) are developed in the most economically viable regions of the country.
To support decision making by policymakers and investors on the location of new infrastructure and hydrogen hubs in Australia, Geoscience Australia, in collaboration with Monash University, has developed an open source software platform, known as Bluecap, to estimate the regional economic potential for resource development in Australia (Walsh et al., 2020; 2021). Originally created to determine regional potential for mineral projects, the software platform has been extended to evaluate the economic potential for renewable hydrogen and hydrogen produced from natural gas and coal with carbon capture and storage.
Bluecap conducts detailed geospatial-financial analyses of future large-scale hydrogen projects and assesses the quality of energy resources (such as wind, photovoltaic, concentrated solar power, or hybrid wind and solar) required to produce hydrogen. It also includes the costs associated with rail and road transportation infrastructures and total transportation distances, pipelines to export ports, distances and costs of water access, and geological hydrogen storage options in the software’s analysis. For blue hydrogen production (i.e., hydrogen produced from natural gas or coal with carbon capture and storage), the model also accounts for the cost of storage and transmission to the closest sequestration site.
Using the Bluecap software, we have modelled the Net Present Value (NPV) of producing, transporting, and geologically storing renewable and CCS hydrogen across Australia in 2030. Our work integrates the economics of geological hydrogen storage with the hydrogen supply chain for the first time in the Australian context, providing novel insight into the economic possibility of Australia succeeding in its ambition of leading the global hydrogen market by 2030.
Integration of Geological Hydrogen Storage Into Bluecap
The original hydrogen model in Bluecap allowed for the assessment of hydrogen sold either at the “plant-gate” or for export (cf. Walsh et al., 2021). The first option considers the sale of produced hydrogen at the site of production, assessing the NPV of a potential project where hydrogen transport costs are not considered. The second option assumes produced hydrogen is transported to the nearest suitable export location, assessing the impact hydrogen transport costs have on the NPV of a potential project.
The key steps involved in the Bluecap calculation are illustrated in Figure 1. First a local plant model is used to calculate the year on year cash flow for the project for a given hydrogen production target and fixed capacity factor. The plant model accounts for the startup and ongoing costs of both the renewable energy source and the cost of electrolysis, as well as any state or federal taxes or rebates on the project. Next the plant cost estimates are repeated for different capacity factors to build up a lookup function describing the plant costs. These lookup functions are then applied to Australia-wide capacity factor maps, to obtain regional estimates of the plant costs. These costs are combined with estimates of the associated infrastructure and transportation costs derived from maps of the local infrastructure distances: distance to roads, rail, and export ports, transportation distances, and distances to water and power transmission (if required).
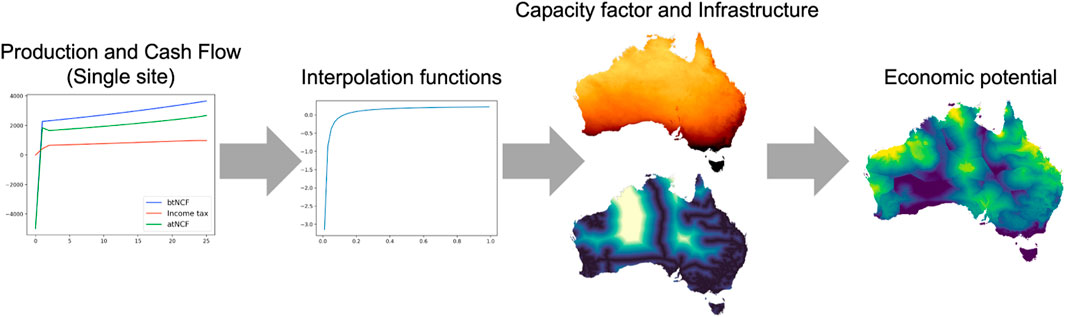
FIGURE 1. Key steps involved in the Bluecap hydrogen storage calculation: A model of the plant specific cost is first used to determine the year on year cash flow for a given production target; the model is used to create interpolation functions relating the plant costs to the underlying renewable resource; the interpolation functions is combined with maps of the associated renewable energy resource and available infrastructure to generate maps of regional economic potential.
In the present extension to the Bluecap code, we have added geological hydrogen storage as a supplementary option under the “hydrogen-end point” selection. Produced hydrogen my be sold to a salt storage facility (i.e., salt cavern) or depleted gas field facility, at a discount equivalent to the levelised cost of storage (LCoS) as indicated in Table 1. This allows the user to assess the impact of hydrogen transport costs and levelised cost of geological storage on the NPV of a potential hydrogen project—an assessment that has not yet been conducted in the Australian context.
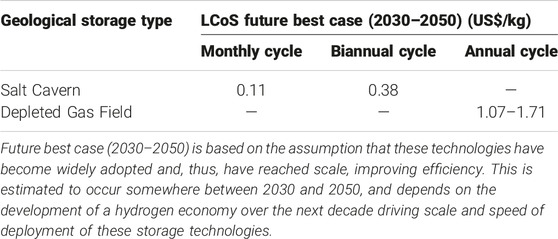
TABLE 1. BNEF (2020) future estimates on levelised cost of hydrogen storage (LCoS) ($/kg of working capacity of hydrogen) in salt caverns and depleted gas fields.
Geological Storage Cost Estimates
BNEF (2020) provides the default cost estimates used in our model to map the economic potential of hydrogen production paired with geological storage up to 2030. The complexity of determining costs for geological hydrogen storage arises from the extensive variations in factors such as storage capacities, operating conditions, and the frequency of injection and withdrawal cycles, which is beyond the scope of this study. For consistency, the default estimates used in our model draw primarily from a single, comprehensive source (BNEF, 2020), which takes into account a wide range of assumptions related to the unique characteristics of both salt caverns and depleted gas fields. These projected cost estimates align with those suggested by the CSIRO National Hydrogen Roadmap (Bruce et al., 2018b) for salt caverns (in the best-case scenario with monthly cycling, a storage capacity of 1.8 million cubic meters has a levelized cost of US$0.11–0.14 per kg) and Yousefi et al. (2023) for depleted gas fields. These default values, however, can be overridden by the user’s own cost models as desired.
Table 1 outlines the BNEF (2020) levelised cost of storage (LCoS) estimates for both salt cavern and depleted gas field hydrogen storage. Capex, opex and cycling rates are included in the estimates. For annual salt cavern storage, we use an estimated cost of 0.70 US$/kg, which is calculated from the monthly and biannual cycle costs assuming a linear relationship between cost and storage duration.
The cost of cycling hydrogen gas in and out of caverns and depleted fields is also included in the LCoS. Cycling rates impact capex and opex, and are a key determinant of overall storage costs. For salt caverns, monthly and bi-annual cycling rates are based on an assumed cycling time of 20 days fill time and 10 days withdrawal time of working gas. Depleted gas fields are assumed to cycle once per year. Maximum gas injection and withdrawal rates in porous reservoirs are significantly less than those in open salt caverns, impacting the rate of cycling (maximum 0.5%–1% daily withdrawal, or roughly 1–2 cycles per year; BNEF, 2020).
Hydrogen transport costs from a production facility to the closest potential export location, or geological storage site, were also considered in the model. Hydrogen transportation costs by pipeline are based on estimates for Australia (Kan and Shibata, 2018) that include both capital and operational cost-estimates. Road and rail transportation costs are based on estimates provided by the CSIRO for fleet capex and opex (Bruce et al., 2018a) and AusIMM estimates for new road or rail construction (Burt et al., 2012). A detailed description on how the Bluecap software conducts hydrogen transport assessments on a regional scale and how suitable locations for hydrogen export have been determined can be found in Walsh et al. (2021).
Geological Storage Locations
Here, we describe the model to calculate the cost of transporting hydrogen from a potential production location to a geological storage site. The ability to utilise a specific location for large-scale hydrogen storage—as an underground salt cavern or depleted gas field—is highly dependent on favourable geology (Sadler et al., 2016; Bruce et al., 2018a). As such, only a select number of potential geological storage sites across Australia are considered in this study.
Salt Cavern Site Selection
Estimates vary as to how thick salt formations should be for large scale storage. For example, Caglayan et al. (2020) estimates that salt formations of 200 m or more are needed for hydrogen storage, while Williams et al. (2022) state that halite formations 50 m or greater may be sufficient. The thickness requirements will also vary depending on whether the cavern is located within a salt dome or bedded salt layer (Caglayan et al., 2020; Bradshaw et al., 2023). For the purpose of this study we assume a minimum thickness of 100 m is required for long-term storage. Figure 2 shows the locations of halite (salt) bearing basins in Australia, and the known, thick (
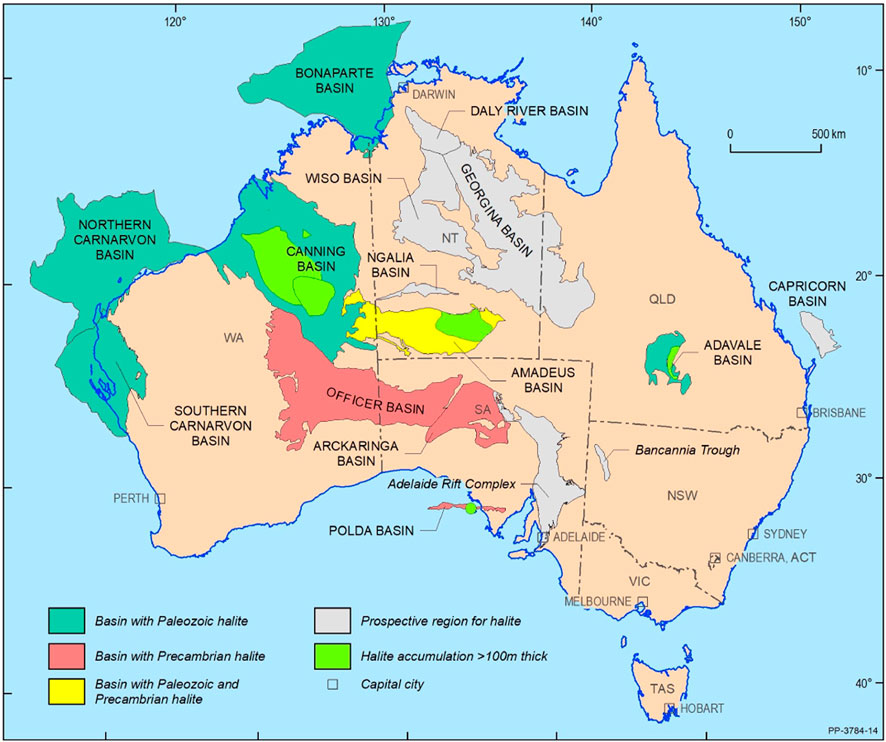
FIGURE 2. Map showing locations of halite (salt) bearing basins across Australia and known, thick (
A spatial dataset of the locations of thick halite formations (lime green polygons in Figure 2) were included in Bluecap to model the economic potential of coupling a potential hydrogen project with a potential salt cavern storage site. This dataset was created by Geoscience Australia and can be freely accessed at AusH2 (Geoscience Australia, 2021c).
Depleted Gas Field Site Selection
Figure 3 shows the locations of conventional onshore depleted gas fields and underground gas storage facilities (depleted gas fields that have been repurposed to store seasonally store natural gas) in Australia. Offshore depleted gas fields have not been considered as potential hydrogen storage options in our Bluecap modelling due to the increased costs and technical complexity associated with offshore gas storage. Current petroleum titles included in Figure 3 (shown in red) highlight operating petroleum fields that could potentially be repurposed for hydrogen storage once depleted.
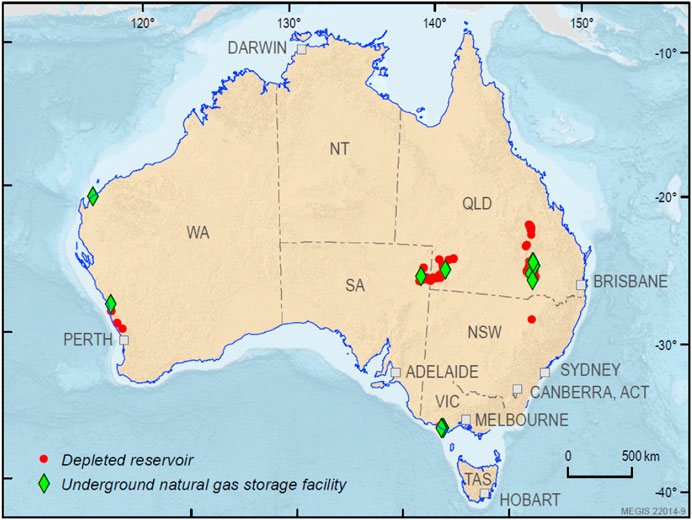
FIGURE 3. Map showing locations of conventional onshore depleted gas fields and underground gas storage facilities in Australia. Current petroleum production title areas are also included to highlight currently operating petroleum fields that could potentially, in the future, be repurposed for hydrogen storage once depleted.
The data points for onshore depleted gas fields were collated from a range of sources including data provided directly to Geoscience Australia from State Geological Surveys (South Australian, Victorian and Western Australian data points), Australian Stock Exchange (ASX) reports (New South Wales data points), and open access data from the Queensland Government’s Open Data Portal (Queensland data points). Using the Queensland data portal, we determined if a gas field located in Queensland was depleted by cross referencing six-monthly gas production data with gas reserves statistics over a four and half year period (30 June 2015 to 31 December 2019). If a field reported zero million cubic metres for both “total gas produced” and “remaining reserves” as at 31 December 2019, the field was classified as depleted and included in the Bluecap modelling.
The location data for underground gas storage facilities were collated from a range of open source websites and reports—including works by Core Energy Group (2015)) for the Australian Energy Market Operator (AEMO) on gas storage facilities in eastern and south-eastern Australia—ASX reports, and storage company reports. Similar to the halite formations, a spatial dataset of the locations of onshore depleted gas fields and underground gas storage facilities was included in Bluecap to model the economic potential of coupling a potential hydrogen project with a depleted gas field storage site. This dataset was created by Geoscience Australia and can be freely accessed at AusH2 (Geoscience Australia, 2021a).
Results and Discussion
In this section, we consider two main scenarios for hydrogen storage in 2030 using the Bluecap modelling tools. The first examines the potential for using hydrogen produced from renewable energy sources, while the second evaluates potential hydrogen production from natural gas with carbon capture and storage. Using the Bluecap modelling tools, we consider the impact that salt cavern and depleted gas field hydrogen storage has on the NPV of potential hydrogen operations across Australia.
2030 Renewable Hydrogen Scenario
In this section, we consider the impact of potential hydrogen storage facilities on the production of green hydrogen (i.e., hydrogen produced from 100% renewable energy) using solar power. Table 2 shows the main assumptions used to model the 2030 renewable hydrogen scenario. The LCoS estimates for salt cavern and depleted gas field storage are optimistic, assuming the cheapest end of the future best case estimates by BNEF (2020) are achieved by 2030.
The simulation results are presented in Figure 4. Figure 4A maps the estimated NPV of hydrogen projects considering both sales to export ports and hydrogen storage facilities, while Figure 4B considers the sale of hydrogen to storage locations only. Both maps show regions of positive NPV around the key storage sites, with gradations away from those locations caused by the effect of the cost of hydrogen-transportation and access to road and rail infrastructure.
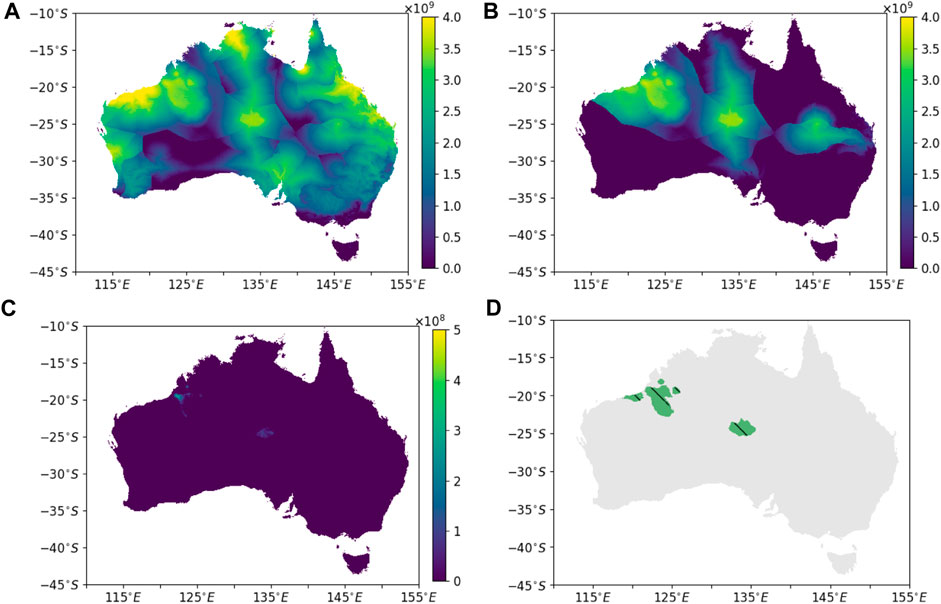
FIGURE 4. Modelled regional NPV for a 1,000 tonne per day renewable (i.e., green) hydrogen plant using solar electricity in 2030: (A) hydrogen transported to either a potential export or a storage location; (B) salt cavern storage only; and (C) salt cavern storage with a 12 months cycle. The final map (D) highlights positive NPV regions in the 95% percentile for storage. Hydrogen is produced through PEM electrolysis (estimated capex US$440/kW in 2030) with hydrogen transport via pipeline to the nearest suitable export location or geological storage site. The target hydrogen price is $3.10/kgH2. Areas of greater NPV indicate more favourable conditions for hydrogen production under these assumptions.
While sales to salt cavern storage remain competitive with export port sales under the assumptions listed in Table 2, the higher cost of depleted gas storage meant that these locations did not report a positive NPV. Nevertheless there may be other reasons to consider development of depleted gas reservoirs, as discussed further in Storage in Depleted Gas Reservoirs section. We also consider the effect of storage duration on the potential for salt storage, using an annual cycle in Figure 4C. While the economic potential is considerably reduced under these assumptions, areas of positive NPV can still be found in the Pilbara and central Australia. Both regions are further highlighted when we outline the high potential regions for storage in Figure 4D.
2030 CCS Hydrogen Scenario
Table 3 shows the main assumptions used to model the 2030 CCS hydrogen scenario. The natural gas price has been forecast at AU$10.00/GJ in line with estimates by AEMO (2022). It should be noted that due to the elevated price of natural gas, the model does not predict any regions of positive NPV at $3.10/kgH2 with or without hydrogen storage. Nevertheless, the gas price is extremely volatile due to the ongoing war in Ukraine and uncertainties around future gas supply and demand. As such, the target hydrogen price has also been increased in these models to $4.20/kgH2. As in the renewable energy scenario, the LCoS estimates for salt cavern and depleted gas field storage are optimistic, assuming the least-expensive future best case estimates by BNEF (2020) are achieved by 2030.
The blue hydrogen model favours hydrogen salt cavern storage in the Adavale Basin, due to its proximity to the natural gas network and likely CCS reservoirs (Figure 5). Again, despite their close proximity to the natural gas network, hydrogen storage in natural gas reservoirs was not found to be competitive with sales to either salt cavern storage or directly to export ports.
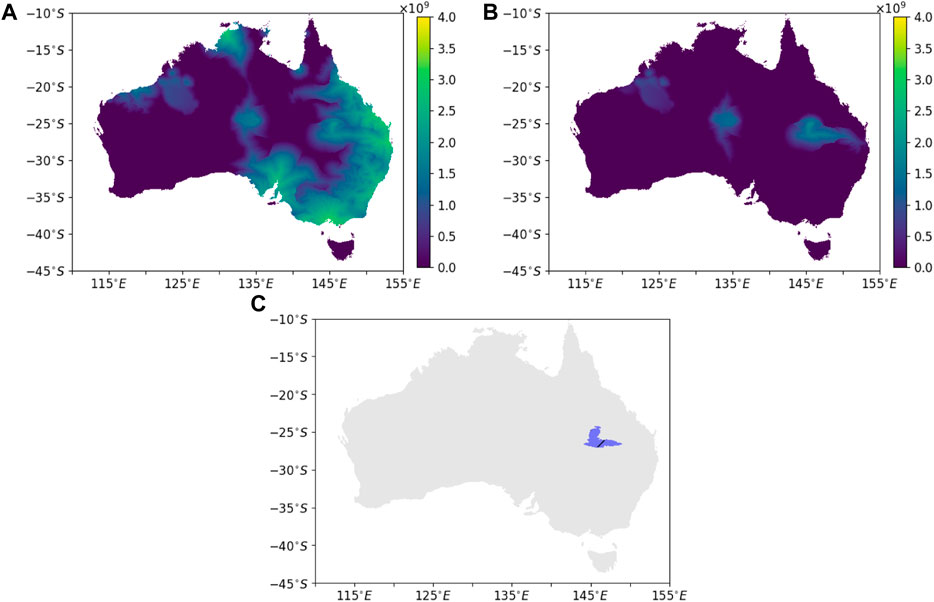
FIGURE 5. Modelled regional NPV for a 1,000 tonne per day CCS (i.e., blue) hydrogen plant using natural gas in 2030: (A) hydrogen transported to either a potential export or a storage location; (B) salt cavern storage only. The lower map (C) highlights positive NPV regions in the 95% percentile for storage. Hydrogen is produced via steam methane reformation and CCS with hydrogen transport via pipeline to the nearest suitable export location or geological storage site. The forecasted gas price for 2030 is AU$10.00/GJ (AEMO, 2022) and the target hydrogen price is $4.20/kgH2. Areas of greater NPV indicate more favourable conditions for hydrogen production under these assumptions.
Storage in Depleted Gas Reservoirs
Hydrogen storage in depleted gas reservoirs is anticipated to be significantly more expensive than salt cavern storage on a per kilogram basis, due primarily to the low cycling rates, need for cushion gas and greater losses experienced in gas reservoirs (Bruce et al., 2018a). Under the assumptions adopted in this paper, the increased cost of storage in depleted gas reservoirs meant that no sites could be found on par with production of hydrogen for direct export. Nevertheless, there may be other reasons to consider storage of hydrogen in depleted gas reservoirs; for example, for long-term energy security or in anticipation of decreased storage costs in the future.
Accordingly, Figure 6 highlights the regions best suited for hydrogen production and depleted gas reservoir storage under both the renewable and CCS scenarios. For the purpose of this figure, we have set the storage cost to 0, to highlight the best-performing locations. As was the case for salt cavern storage, the northwestern fields tend to favour hydrogen storage from renewable energy sources. Fields in central Queensland and at the Queensland/South Australian border are highly ranked under both scenarios. Locations on the South coast of Victoria are favoured for storage of hydrogen from natural gas with CCS, due to the proximity of the gas network and the Otway CO2 storage basin.
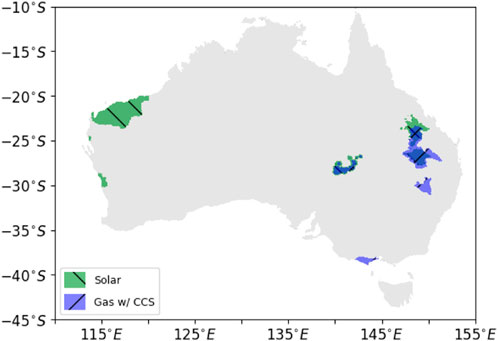
FIGURE 6. Locations best suited (95th percentile) for hydrogen production with subsequent storage in depleted gas reservoirs under the renewable energy scenario (green) and the CCS scenario (blue).
Conclusion
Australia hosts significant capacity for geological hydrogen storage either in the form of salt cavern storage or depleted gas fields. Known halite accumulations greater than 100 m in thickness are present in the Canning Basin in Western Australia, the Amadeus Basin in the Northern Territory and the Adavale Basin in Queensland respectively, while natural gas storage sites, depleted and current reservoirs exist in all Australian states and territories except Tasmania.
The suitability of sites for hydrogen storage will depend on their proximity to potential sites for hydrogen production and the type and cost of storage. To assess the potential impact of these sites, we have developed a model to estimate the regional economic potential for hydrogen production and storage. The module assesses the cost of producing and transporting hydrogen either to an export location or a potential storage site, and can be used to evaluate the potential benefit for selling hydrogen to each destination.
The results from the analysis illustrate that salt cavern formations could provide potential benefit for hydrogen production, particularly around the Pilbara region in north Western Australia. This is due to the high potential for solar production in this region and the relatively low cost of salt cavern storage. While depleted gas fields are naturally closer to sites of gas production and transmission infrastructure, the current high prices for natural gas, the high cost of storage and low cycling rates in depleted gas reservoirs makes them less economically favourable.
Data availability statement
The datasets presented in this study can be found in online repositories. The names of the repository/repositories and accession number(s) can be found below: https://portal.ga.gov.au/persona/hydrogen.
Author Contributions
SW—Simulation, writing, editing. LE—Writing, research, analysis. CW—Editing. AF—Editing, project management. All authors contributed to the article and approved the submitted version.
Funding
This study was completed under the Australian Government’s Exploring for the Future program. AF publishes with the permission of the CEO of Geoscience Australia.
Conflict of Interest
The authors declare that the research was conducted in the absence of any commercial or financial relationships that could be construed as a potential conflict of interest.
Publisher’s Note
All claims expressed in this article are solely those of the authors and do not necessarily represent those of their affiliated organizations, or those of the publisher, the editors and the reviewers. Any product that may be evaluated in this article, or claim that may be made by its manufacturer, is not guaranteed or endorsed by the publisher.
Footnotes
1The online version of the HEFT tool is available at https://portal.ga.gov.au/persona/heft
2The Bluecap repository is located at https://github.com/GeoscienceAustralia/bluecap
References
AEMO (2022). 2022 Integrated System Plan (ISP). Available from: https://aemo.com.au/en/energy-systems/major-publications/integrated-system-plan-isp (Accessed December, 2022).
Amid, A., Mignard, D., and Wilkinson, M. (2016). Seasonal Storage of Hydrogen in a Depleted Natural Gas Reservoir. Int. J. hydrogen energy 41 (12), 5549–5558. doi:10.1016/j.ijhydene.2016.02.036
Andersson, J., and Grönkvist, S. (2019). Large-scale Storage of Hydrogen. Int. J. hydrogen energy 44 (23), 11901–11919. doi:10.1016/j.ijhydene.2019.03.063
BNEF (2020). The Economics of Storage – Storing Clean Molecules at Scale. Tech. rep. Bloomberg New Energy Finance: Bloomberg.
Bouckaert, S., Pales, A. F., McGlade, C., Remme, U., Wanner, B., Varro, L., et al. (2021). Net Zero by 2050: A Roadmap for the Global Energy Sector.
Bradshaw, M., Rees, S., Wang, L., Szczepaniak, M., Cook, W., Voegeli, S., et al. (2023). Australian Salt Basins–Options for Underground Hydrogen Storage. APPEA J. 63 (1), 285–304. doi:10.1071/aj22153
Bruce, S., Temminghoff, M., Hayward, J., Schmidt, E., Munnings, C., Palfreyman, D., et al. (2018a). National Hydrogen Roadmap. Australia: CSIRO.
Bruce, S., Temminghoff, M., Hayward, J., Schmidt, E., Munnings, C., Palfreyman, D., et al. (2018b). National Hydrogen Roadmap. Australia: CSIRO.
Bunger, U., Michalski, J., Crotogino, F., and Kruck, O. (2016). “Large-scale Underground Storage of Hydrogen for the Grid Integration of Renewable Energy and Other Applications,” in Compendium of Hydrogen Energy. Woodhead Publishing Series in Energy. Editors M. Ball, A. Basile, and T. N. Veziroğlu (Oxford: Woodhead Publishing), 133–163. doi:10.1016/B978-1-78242-364-5.00007-5
Caglayan, D. G., Weber, N., Heinrichs, H. U., Linßen, J., Robinius, M., Kukla, P. A., et al. (2020). Technical Potential of Salt Caverns for Hydrogen Storage in Europe. Int. J. Hydrogen Energy 45 (11), 6793–6805. doi:10.1016/j.ijhydene.2019.12.161
Chen, F., Ma, Z., Nasrabadi, H., Chen, B., Saad Mehana, M. Z., Van Wijk, J., et al. (2023). Capacity Assessment and Cost Analysis of Geologic Storage of Hydrogen: A Case Study in Intermountain-West Region USA. Int. J. Hydrogen Energy 48 (24), 9008–9022. doi:10.1016/j.ijhydene.2022.11.292
Core Energy Group (2015). Gas Production and Transmission Costs Eastern and South Eastern Australia. Tech. rep. Core Energy Group.
Craig, A., Newman, S., Stephenson, P., Evans, C., Yancazos, S., and Barber, S. (2022). Hydrogen Storage Potential of Depleted Oil and Gas Fields in Western australia. APPEA J. 62 (1), 185–195. doi:10.1071/aj21146
DCCEEW (2022). State of Hydrogen 2022. Technical Report. Australia: Department of Climate Change and Energy Efficiency. Available at: https://www.dcceew.gov.au/sites/default/files/documents/state-of-hydrogen-2022.pdf (Accessed December, 2022).
DISER (2021). Growing australia’s Hydrogen Industry. Tech. rep. Australian Government: Department of Industry, Science, Energy and Resources.
Ennis-King, J., Michael, K., Strand, J., Sander, R., and Green, C. (2021). Underground Storage of Hydrogen: Mapping Out Options for australia. Future Fuel CRC.
Geoscience Australia (2021a). AusH2 - Australia’s Hydrogen Opportunities Tool. URL Available from: https://portal.ga.gov.au/persona/hydrogen (Accessed December, 2022).
Geoscience Australia (2021b). Geoscience australia’s Submission to Nsw Legislative Council’s Standing Committee on State Development’s Inquiry into Development of a Hydrogen Industry in New South Wales, Submission 4. Tech. rep. URL Available from: https://www.parliament.nsw.gov.au/lcdocs/submissions/70653/0004%20Geoscience%20Australia.pdf (Accessed December, 2022).
Geoscience Australia (2021c). The Hydrogen Economic Fairways Tool (HEFT). URL Available from: https://portal.ga.gov.au/persona/heft (Accessed December, 2022).
Haines, P. (2010). The Carribuddy Group and Worral Formation, Canning Basin, Western Australia: Reassessment of Stratigraphy and Petroleum Potential. APPEA J. 50 (1), 425–444. doi:10.1071/aj09026
Hashimoto, T., Bailey, A., Chirinos, A., and Carr, L. (2018). Onshore Basin Inventory Volume 2: The Canning, Perth and Officer Basins. Tech. rep. Geoscience Australia. doi:10.11636/Record.2018.018
Hevin, G. (2019). “Underground Storage of Hydrogen in Salt Caverns,” in European Workshop on Underground Energy Storage November 7th–8th.
IEA (2019). The Future of Hydrogen: Seizing Today’s Opportunities. Tech. rep. International Energy Agency.
IRENA (2021). World Energy Transition Outlook 2021. Tech. rep. International Renewable Energy Agency.
Judd, R., and Pinchbeck, D. (2016). “Hydrogen Admixture to the Natural Gas Grid,” in Compendium of Hydrogen Energy (Elsevier), 165–192.
Kan, S., and Shibata, Y. (2018). “Evaluation of the Economics of Renewable Hydrogen Supply in the Apec Region,” in 34th Conference on Energy, Economy, and Environment (Japan Society of Energy and Resources (JSER).
K. Burt, C. Lockyer, K. McShane, and O. T. Fong (Editors) (2012). Cost Estimation Handbook. 2nd ed. (Australasian Inst. of Mining and Metallurgy). Monograph 27.
Khaledi, K., Mahmoudi, E., Datcheva, M., and Schanz, T. (2016). Stability and Serviceability of Underground Energy Storage Caverns in Rock Salt Subjected to Mechanical Cyclic Loading. Int. J. Rock Mech. Min. Sci. 86, 115–131. doi:10.1016/j.ijrmms.2016.04.010
Kobos, P. H., Lord, A. S., Borns, D. J., and Klise, G. T. (2011). A Life Cycle Cost Analysis Framework for Geologic Storage of Hydrogen: A User’s Tool. Tech. rep. Albuquerque, NM, and Livermore, CA: Sandia National Laboratories (SNL).
Kruck, O., Crotogino, F., Prelicz, R., and Rudolph, T. (2013). Overview on All Known Underground Storage Technologies for Hydrogen. Project HyUnder–Assessment of the Potential, the Actors and Relevant Business Cases for Large Scale and Seasonal Storage of Renewable Electricity by Hydrogen Underground Storage in Europe. Report D 3.
Lord, A. S., Kobos, P. H., and Borns, D. J. (2014). Geologic Storage of Hydrogen: Scaling up to Meet City Transportation Demands. Int. J. Hydrogen Energy 39 (28), 15570–15582. doi:10.1016/j.ijhydene.2014.07.121
Makridis, S. (2016). “Hydrogen Storage and Compression,” in Methane and Hydrogen for Energy Storage. Editor R. D. S. T. Carriveau (IET Digital Library).
Muhammed, N. S., Haq, B., Al Shehri, D., Al-Ahmed, A., Rahman, M. M., and Zaman, E. (2022). A Review on Underground Hydrogen Storage: Insight into Geological Sites, Influencing Factors and Future Outlook. Energy Rep. 8, 461–499. doi:10.1016/j.egyr.2021.12.002
Sadler, D., Cargill, A., Crowther, M., Rennie, A., Watt, J., Burton, S., et al. (2016). H21 Leeds City Gate. Leeds City Gate, Northern Gas Networks, Wales and West Utilities 1325.
Simon, J., Férriz, A. M., and Correas, L. C. (2015). Hyunder–hydrogen Underground Storage at Large Scale: Case Study spain. Energy procedia. 73, 136–144. doi:10.1016/j.egypro.2015.07.661
Tarkowski, R. (2019). Underground Hydrogen Storage: Characteristics and Prospects. Renew. Sustain. Energy Rev. 105, 86–94. doi:10.1016/j.rser.2019.01.051
Walsh, S. D., Easton, L., Weng, Z., Wang, C., Moloney, J., and Feitz, A. (2021). Evaluating the Economic Fairways for Hydrogen Production in australia. Int. J. Hydrogen Energy 46 (73), 35985–35996. doi:10.1016/j.ijhydene.2021.08.142
Walsh, S. D., Northey, S. A., Huston, D., Yellishetty, M., and Czarnota, K. (2020). Bluecap: A Geospatial Model to Assess Regional Economic-Viability for Mineral Resource Development. Resour. Policy 66, 101598. doi:10.1016/j.resourpol.2020.101598
Wells, A. T., and Hodgson, I. (1980). Evaporites in australia. Evaporites in Australia Australian Government Publishing Service.
Williams, J. D., Williamson, J., Parkes, D., Evans, D. J., Kirk, K. L., Sunny, N., et al. (2022). Does the united kingdom Have Sufficient Geological Storage Capacity to Support a Hydrogen Economy? Estimating the Salt Cavern Storage Potential of Bedded Halite Formations. J. Energy Storage 53, 105109. doi:10.1016/j.est.2022.105109
Yousefi, S. H., Groenenberg, R., Koornneef, J., Juez-Larré, J., and Shahi, M. (2023). Techno-economic Analysis of Developing an Underground Hydrogen Storage Facility in Depleted Gas Field: A Dutch Case Study. Int. J. Hydrogen Energy. doi:10.1016/j.ijhydene.2023.04.090
Keywords: green hydrogen, blue hydrogen, energy transition, geological storage, economic fairways
Citation: Walsh SDC, Easton L, Wang C and Feitz AJ (2023) Evaluating the Economic Potential for Geological Hydrogen Storage in Australia. Earth Sci. Syst. Soc. 3:10074. doi: 10.3389/esss.2023.10074
Received: 23 December 2022; Accepted: 19 July 2023;
Published: 31 July 2023.
Edited by:
Mark Thomas Ireland, Newcastle University, United KingdomReviewed by:
Adnan Aftab, Curtin University, AustraliaAlex Bump, The University of Texas at Austin, United States
Johannes M. Miocic, University of Groningen, Netherlands
Copyright © 2023 Walsh, Easton, Wang and Feitz. This is an open-access article distributed under the terms of the Creative Commons Attribution License (CC BY). The use, distribution or reproduction in other forums is permitted, provided the original author(s) and the copyright owner(s) are credited and that the original publication in this journal is cited, in accordance with accepted academic practice. No use, distribution or reproduction is permitted which does not comply with these terms.
*Correspondence: Stuart D. C. Walsh, stuart.walsh@monash.edu