- 1Department of Earth and Environmental Science, University of Minnesota, Minneapolis, MN, United States
- 2Department of Physics and Astronomy, Colgate University, Hamilton, NY, United States
- 3Minnesota Geological Survey, University of Minnesota, St. Paul, MN, United States
Chloride concentrations in surface and groundwater within the Twin Cities Metropolitan Area of Minnesota are increasing and hand-sample monitoring is not sufficient to understand the scale and temporal nuance of chloride concentrations within the aquifer system. We explore the relationships between chloride concentration and specific conductance (SC) in regional aquifers as a mechanism to generate a larger quantity of data related to groundwater chloride concentrations at a higher temporal frequency. Paired measurements (N = 2,118) from 1,050 wells collected between 1972 and 2022 allow statistical relationships to be generated for several aquifer units that enable the use of SC as either a direct proxy or qualitative indicator of chloride concentration going forward. In the uppermost unconsolidated Quaternary aquifer and in the immediately underlying bedrock aquifer (Platteville Formation), correlation between chloride concentration and specific conductance imply that conductances of 1,350 and 3,800 μS/cm correspond to state chronic and acute exposure standards of 230 mg/L and 860 mg/L, respectively. SC values have increased through time in bedrock aquifers that provide the region’s largest volume of residential and industrial groundwater (the Prairie du Chien and Jordan aquifers) and interpreting these changes in detail requires consideration of the local hydrogeologic context.
Introduction
In the Twin Cities Metropolitan Area (TCMA) chloride concentrations are increasing in several regional aquifers due to the use of rock salt (NaCl) as a road deicer over the last half century (McDaris et al., 2022). High groundwater chloride concentrations can affect the taste of drinking water, limit the utility of the water for agricultural and industrial use, contribute to corrosion of built infrastructure, and harm freshwater ecosystems. Although most measurements of groundwater chloride concentration remain below chronic environmental exposure limits set by state and federal regulators, continued increases will pose environmental quality challenges and eventually require expensive mitigation strategies (e.g., reverse osmosis filtration and the drilling of new wells) for the 75% of Minnesotans who get their drinking water from groundwater aquifers via residential or municipal wells. Understanding the mechanisms by which chloride concentrations are changing in groundwater is vitally important to the region’s environmental and economic future.
Identifying mechanisms and rates of movement for chloride will require large data sets at high temporal frequencies. Yet the time-intensive nature of hand sampling, combined with staffing limitations at most state and federal agencies with groundwater oversight authority, places limits on the data resolution that can be achieved through current groundwater monitoring practices. The available data consist of highly detailed geochemical analyses gathered annually in a small fraction of available wells. Under this sampling regimen, sub-annual oscillations are invisible and long-term trends can be obscured depending on the precise timing of sampling. Specific conductance (SC) measurements, which are comparatively inexpensive and easy to automate, have the potential to provide new information about chloride and other dissolved constituents of groundwater on extremely fast timescales. Such data could be instrumental in helping monitoring agencies prioritize their limited human resources in a more strategic manner.
This study takes advantage of detailed geologic mapping, high quality drilling records, and well construction information available for the TCMA to determine in which hydrogeologic settings SC can be a useful proxy for chloride concentration. Examining over 50 years of historical data from a number of TMCA aquifers in variable settings, we explore: (1) the relationship between SC and chloride concentration for each aquifer, (2) how the hydrogeologic setting affects the SC-chloride relationship in particular aquifers, and (3) how relative changes in SC over time can be linked to changes in chloride concentration. We demonstrate that SC can be used to directly estimate groundwater chloride concentrations in some aquifers under particular hydrogeologic conditions, and that in some other aquifers relative SC changes over time can also be used to infer changes in chloride concentration.
Hydrogeologic Context
The TCMA basin is comprised of Paleozoic sedimentary bedrock deposited on a shallow marine shelf, overlain by varying thicknesses of Quaternary glacial deposits (Figure 1). Regionally, there are six major bedrock aquifer units dominated by fine-to coarse-grained sandstones or carbonate rocks with extensive secondary porosity (Runkel et al., 2003; Runkel et al., 2006). The shape of the basin allows individual bedrock units to be overlain in some places by other bedrock units and elsewhere to subcrop immediately beneath Quaternary deposits. The composition of those Quaternary deposits also varies greatly across the region. The clay-rich tills of the Des Moines Lobe in the west (commonly exceeding 30 m in thickness) and the patchier, sandier, and thinner tills in the east constitute very different barriers to the infiltration of surface water (Tipping, 2012). The heterogeneous nature of the Quaternary deposits and the extensive secondary pore networks play an important role in the movement of water from the land surface to depth (Runkel et al., 2018).
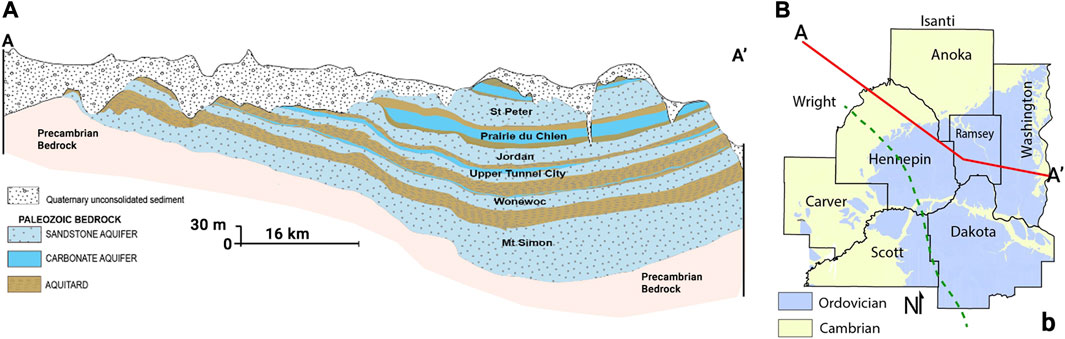
FIGURE 1. Hydrogeologic context of the Twin Cities Metropolitan Area (TCMA). (A) Cross-section view showing the major aquifer units in the region. (B) Map view of the 7 county Twin Cities Metropolitan Area (TCMA). The red line is the path of the cross-section through the region. The dashed green line indicates the easternmost extent of the Des Moines Lobe glacial tills (Adapted from McDaris et al., 2022).
Previous Work
The natural background chloride concentration in Minnesota groundwater is typically less than 7 mg/L (Kroening and Ferrey, 2013). While there are several potential sources for elevated chloride concentrations in groundwater, two main contributors have been identified in Minnesota: road deicing salt and brines from water softeners (MPCA, 2016). More than three-quarters of the total volume of water softener brine passes into rivers via sanitary sewers and water treatment plants (Overbo et al., 2019). By contrast, road salts are distributed directly into the environment where they have led to elevated salinity in TCMA lakes (Novotny et al., 2008). Because surface water and groundwater are intimately connected, this excess chloride is now present in the Quaternary and bedrock aquifers of the region (McDaris et al., 2022).
Specific conductance (SC) is the capacity of an aqueous solution to conduct electricity normalized to a standard temperature of 25°C, and empirically it is proportional to the concentration of total dissolved solids present (Chang et al., 1983; Hem, 1989; Hayashi, 2004; Fondriest Environmental, Inc, 2014). Since SC is determined by contributions from all dissolved ions, it provides an aggregate measure of water quality.
SC is often measured during manual stream and groundwater monitoring efforts and is useful for basic water quality assessments. The World Health Organization (2004) has set a recommendation for the upper limit of SC for drinking water (400 μS/cm). The US EPA developed a benchmark methodology for protecting aquatic life in surface waters based on SC (Cormier et al., 2011). Elevated SC (greater than 600 μS/cm) is one of the criteria for the Minnesota Department of Health in determining whether a given aquifer is highly sensitive to potential pollution (MDH, 2016). Aquifers that have been contaminated with hydrocarbons exhibit a distinctive relationship between total dissolved solids and bulk SC (Atekwana et al., 2004).
Continuous, automated SC measurements have not been as widely utilized in groundwater monitoring, though such measurements are used extensively in studies of seawater intrusion into coastal aquifers (e.g., Diamantis and Petalas, 1989; Lee et al., 2007; Roehl et al., 2013), exploration of groundwater contaminant build up in agricultural land (e.g., Deverel and Fujii, 1988; Hamilton and Helsel, 1995; Abanyeh et al., 2005), and investigation of groundwater-surface water interactions (e.g., Chen et al., 2006; Fernald and Guldan, 2006; Cox et al., 2007). In the current study, we explore the variations in the relationship between SC and chloride concentration in a well-characterized, multi-aquifer groundwater system across an urban area with decades of intensive road salt application.
The contribution to SC of any single dissolved constituent such as chloride can be determined empirically for a particular water body by analyzing paired measurements of its concentration and the SC of the solution. A robust and statistically significant trend in that relationship can allow the estimation of one from the other (Granato et al., 2015). This correlation is widely used in monitoring surface waters and springs (e.g., Panno et al., 2003; Rasmussen et al., 2005; Zamella et al., 2007; Fisher and Feinberg, 2019; Moore et al., 2020; Yang and Moyer, 2020). In Minnesota, Novotny et al. (2008) established a relationship between SC and chloride concentration for surface waters by sampling 13 lakes around the TCMA:
This work also demonstrated the link between elevated chloride concentrations in lakes and the application of road salt in the winter.
The SC-chloride relationship can also be determined theoretically from first principles. The contribution of each dissolved ionic species to specific conductance is the product of its electric charge, electrophoretic mobility (i.e., the speed at which ions drift through the water per unit electric field), and concentration. For example, chloride ions have a mobility of −7.9 × 10−8 m2 V/s (Aupiais, 2011). If every chloride anion is accompanied by a sodium cation, as in the simple case of halite dissolved in water, then sodium would also contribute to the specific conductance of the water with a mobility of about 5.0 × 10−8 m2 V/s (Lee and Rasaiah, 1996). Increasing the equal concentrations of these two ions in water would increase the specific conductance, with the change in concentration being 0.28 (mg/L)/(μS/cm) times the change in specific conductance. Thus, for a solution of halite dissolved in pure water, we would expect the slope of the SC-chloride relationship to be 0.28. Any replacement of sodium by higher-mobility ions such as potassium (as from water softener brines) or calcium/magnesium (natural components of Minnesota groundwaters), would yield a lower slope (e.g., stoichiometric KCl yields a slope of 0.23 (mg/L)/(μS/cm)).
Methods
Data Sources
The data for this study is derived from McDaris et al. (2021). The data set consists largely of data gathered by three state agencies (Minnesota Pollution Control Agency, Minnesota Department of Natural Resources, and Minnesota Department of Health) spanning 1965–2021. A small number of additional observations were made by McDaris and research scientist Scott Alexander of the University of Minnesota Department of Earth and Environmental Sciences at wells on the University of Minnesota campus. Additional data on the thickness and composition of Quaternary deposits at each well site were obtained from the Minnesota Well Index (MDH, 2023). Since the bulk of the data were gathered as part of monitoring and regulatory work rather than research, a quality control effort was made to remove spurious data points. There are a small number of specific conductance readings from a series of wells taken on the same day or successive days, which are notably out of agreement with previous and/or subsequent readings from these same wells. The geologic conditions and well construction at these sites are not conducive to rapid and significant changes in water chemistry. Instead, the discrepancies seem to indicate a systematic issue with those particular measurements perhaps related to calibration errors or sensor failure. These outliers have been removed from the data set for the purposes of developing chloride-conductance relationships for the different aquifer units. In addition, the original data set contains many measurements of chloride concentration without paired SC measurements which could not be used for the current analysis. After these adjustments to the data set, this study makes use of 2,118 paired measurements from 1,050 wells across 31 aquifer designations (MGS, 2023) between the years of 1972 and 2022. There is a gap from 1986 to 2004, the data from which are presently not available to the public as paper records from state agencies await digitization. The distribution of specific conductance over time in aquifers with more than 100 measurements in the data set is shown in Figure 2.
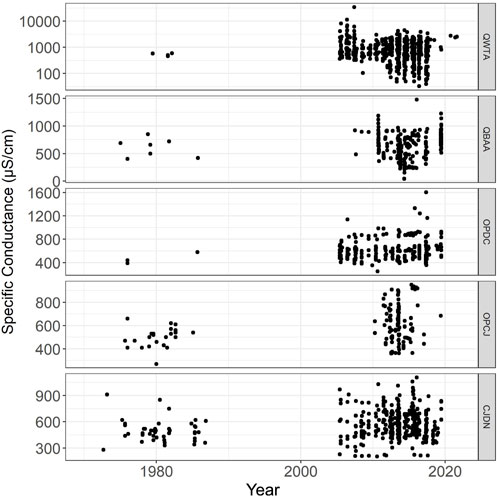
FIGURE 2. Data distributions in time for the units with more than 100 measurements in the dataset in stratigraphic order. The y-axis of the QWTA graph is shown in log scale. All the others are shown in linear scale. Data between 1986 and 2004 were not available.
Computation and Analysis
Data from each aquifer designation was analyzed to determine if there was a significant (p < 0.05) linear correlation between specific conductance and chloride concentration. Parametric linear regressions were performed in RStudio, yielding correlation coefficients (R2) and p values. For the six bedrock aquifers, additional analysis was conducted to ascertain the impacts of geologic context on the observed linear relationships. Data from each unit was classified based on the presence or absence of overlying bedrock layers as well as whether there was more or less than 15 m of clay in the Quaternary deposits at the well site. Again, linear regression models were evaluated for these subpopulations and compared to the bulk unit relationship.
Results and Discussion
Well data from nine aquifer designations showed statistically significant linear relationships between SC and chloride concentration (Table 1). Two of these aquifer designations denote wells that are screened in an interval that spans the boundary between two bedrock aquifers. OPCJ wells span the Prairie du Chien and Jordan units, and CWEC wells span the Wonewoc Sandstone and the Eau Claire Formation. The other seven designations denote wells with screened intervals entirely within a single unit.
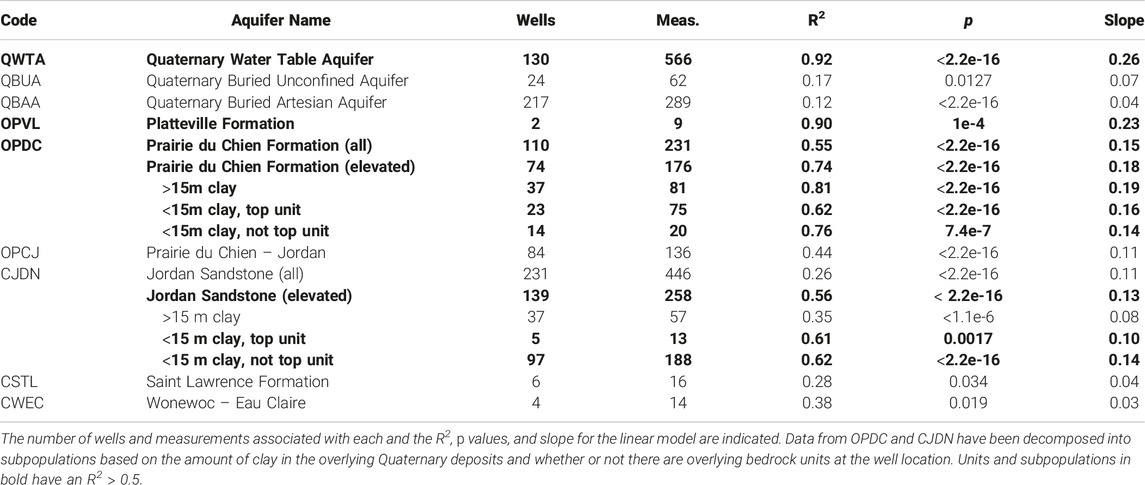
TABLE 1. Units and subpopulations with statistically significant (p < 0.05) linear correlation between specific conductance and chloride concentration.
Of the nine units with significant trends, there are only three with an R2 greater than 0.50 (QWTA, OPVL, and OPDC). These units are discussed in detail below. Three of the bedrock units that did not reach that threshold with all available data had subpopulations of data that did (OPCJ, CJDN, and CSTL). The data for the Jordan Sandstone (CJDN) is explored as an exemplar of these cases. CJDN is also used to demonstrate how to make use of relative changes in SC data over time.
SC-Chloride Relationships
The Quaternary Water Table Aquifer (QWTA) consists of sand and gravel with less than 10 feet of overlying clay-rich confining material (MPCA, 1999). In this unit, we find a robust linear relationship between specific conductance and chloride concentration (Figure 3):
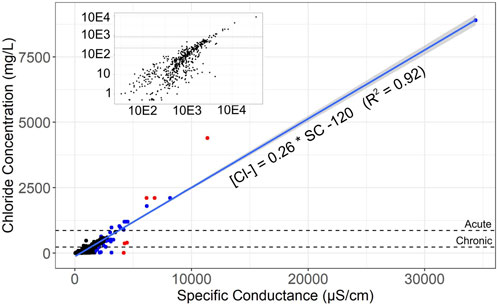
FIGURE 3. Plot of chloride concentration vs. specific conductance in the QWTA unit in the TCMA. Inset shows the same data on a log-log plot to provide greater detail on the data points with lowest chloride concentrations. Dashed lines represent the chronic (230 mg/L) and acute (860 mg/L) environmental water quality standards for chloride set by the state. Red points are those with the most influence on the slope of the best fit line, blue points have moderate influence, and black points have the least influence. The confidence interval for the linear regression is shaded grey.
The similarity of this relationship in the shallow subsurface to that found by Novotny et al. (2008) for surface waters reflects the known, intimate connections between these two reservoirs. Regional lakes and the uppermost water table aquifer often act as a single, interconnected system. (This statistical relationship assumes unique uniform uncertainty in both the specific conductance and chloride concentration measurements. For a more detailed discussion of this assumption, see the Supplementary Information Text S1).
Importantly, the data in Figure 3 are not distributed uniformly on either axis. As a simple exercise to determine the sensitivity of the best-fit line to each individual measurement, we re-fit the ensemble after removing each data point in turn and compared these lines with the fit to the entire dataset. (This procedure was used to demonstrate statistical robustness in a dataset in Anderson et al., 2020.) There are six points (red in Figure 3) whose removal changes the slope of the fit (0.26) by 0.001 or more, and a further 29 points (blue in Figure 3) that affect the slope by 0.0001 or more. Removal of any of the remaining 493 points (black in Figure 3) affects the slope by less than 0.0001. Therefore, despite the nonuniform data distribution, the robustness of this linear relationship is high.
The numerical value of the slope we observe in the relationship between SC and chloride concentration is also very similar to the theoretical calculation above (0.28). Natural water chemistry in most of Minnesota’s aquifers tends to be enriched in calcium and magnesium (Alexander and Alexander, 1989) and any appreciable concentration of these ions could lower the observed slope relative to stoichiometric NaCl. The fact that our empirical slope is close to the value expected from first principles is an a posteriori justification of our assumption of uniform absolute uncertainty in the data.
Under this assumption, Eq. 2 allows the calculation of an expected specific conductance for a given chloride concentration. For instance, the state’s chronic water quality standard for chloride of 230 mg/L would correspond with a specific conductance of 1,350 μS/cm in the QWTA, and the acute standard of 860 mg/L would correspond to a specific conductance of 3,800 μS/cm. To assess the utility of this linear model, we used the specific conductance measurements from wells in the QWTA to “predict” the associated chloride concentration and then compared those predictions with the actual measured chloride concentrations (Figure 4). Though this algorithm can predict values that differ from the measured chloride concentration of some individual well samples by more than 100 mg/L, it correctly characterizes 514 measurements out of 562 (91.5%) as being below, between, or above the state’s chronic and acute water quality standards. Of the 48 mischaracterizations, 38 were chloride overestimations (i.e., the false positive rate was 6.8%), and only 10 were chloride underestimations. The low rate of mischaracterization, and the especially low rate of under-detecting chloride concentrations that exceed the chronic or acute standards, demonstrate that specific conductance measurements can usefully triage wells in the QWTA, and identify those which require follow-up chemical assays to determine if the water is at or above either quality standard.
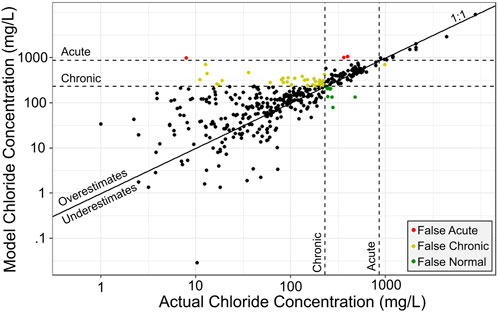
FIGURE 4. Estimation of QWTA chloride concentrations using SC. Vertical lines indicate state chronic (230 mg/L) and acute (860 mg/L) water quality thresholds for chloride. Black points are correctly characterized. Green points were estimated to be below the chronic threshold, but were measured at higher values. Yellow points were estimated to be between the chronic and acute thresholds, but were measured outside this region. Red points were estimated to be above the acute threshold, but were measured at lower values.
Identifying and interpreting chloride-conductance relationships for bedrock aquifers in the TCMA requires closer consideration of the local hydrogeologic context. Wells screened in a single bedrock aquifer can yield waters with greatly variable dissolved constituents that reflect the variable connections to surface water across the extent of the aquifer. For this reason, we have subdivided the data from each unit based first on total overlying clay thickness and then by the unit’s bedrock stratigraphic position. We discuss the relevant bedrock aquifer units from Table 1 in stratigraphic order.
Much of the Platteville Formation (OPVL) has been removed by erosion and its remnants are present as outliers at or near the top of the bedrock sequence in the TCMA. The remnants are heavily fractured and karstified limestone that can produce significant amounts of water.
The data set contained 12 paired measurements of SC and chloride concentration with the OPVL designation, and they come from only two wells. There is little clay in the overlying Quaternary deposits at either site and no intervening bedrock layers. Figure 5 shows the data from this unit along with the trend from the overlying QWTA. It is clear that the OPVL data lie along a slope that is consistent with the downward flow of water from the QWTA into this unit. Given the small number of measurements, this similarity is likely more meaningful than the statistical fit of OPVL data.
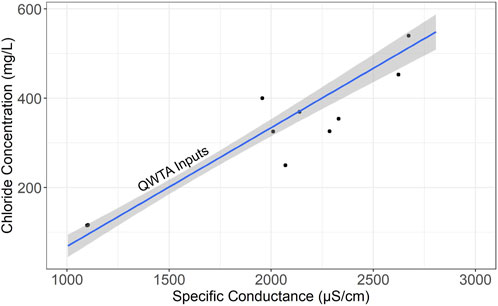
FIGURE 5. Plot of SC vs. chloride concentration data from the OPVL aquifer in the TCMA along with the trend and confidence interval for the overlying QWTA unit.
The Prairie du Chien dolomite (OPDC) underlies the QWTA over much of the region and is an important source of pumped groundwater in the TCMA. The bulk trend of OPDC data is
We see that 76% of OPDC measurements are elevated above the estimated background chloride concentration of 7 mg/L. Focusing only on those data, we see a trend of
To explore whether or not hydrogeologic context could be used to refine this relationship, we subdivided the elevated OPDC data based on how much clay is present in the Quaternary deposits each well site and whether the OPDC was the uppermost bedrock unit at each well site. The measurements are roughly equally split between wells overlain by more and less than 15 m of clay (81 and 95 respectively). Of the elevated measurements from wells with less clay, 75 come from areas where the OPDC is the top bedrock unit and 20 come from areas where it is overlain by at least one other bedrock unit. In the latter setting the OPDC would be confined by the lower St Peter bedrock aquitard. The trends for each of these subpopulations are given here and shown in Figure 6.
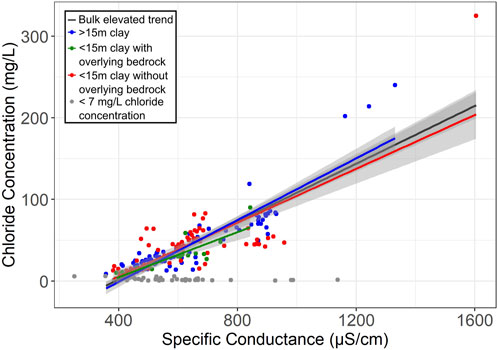
FIGURE 6. SC vs. chloride concentration for the OPDC unit in the TCMA. Grey points are below the estimated background level of 7 mg/L. The black line is the trend of all points above the background threshold. Blue points are wells overlain by >15 m of Quaternary clay. Red points are from wells with <15 m of overlying clay where OPDC is the uppermost bedrock. Green points denote wells were the OPDC is overlain by one or more bedrock aquitards and with <15 m of overlying clay. The confidence interval for each regression is shaded grey. Due to the uncertainty associated with the regression for each subpopulation, it is not possible to distinguish between them statistically.
The confidence intervals on these different trends show significant overlap in the region of the plot where most of the data is found. Unfortunately, this means that knowledge of the hydrogeologic setting cannot be used to improve the estimate of chloride concentration based solely on SC in the OPDC. However, in wells where the chloride concentration is known to be above the natural background, Eq. 6 is a more robust relation than Eq. 5 and should provide a better chloride estimate.
The Jordan Sandstone (CJDN) is the other highly utilized aquifer in the region. Together with the OPDC, the CJDN supplies around 70% of the groundwater pumped in the TCMA (Ross, 2013) and both aquifers already show increased chloride concentrations (McDaris et al., 2022) due to anthropogenic inputs. The CJDN subcrops beneath Quaternary glacial deposits in a crescent around the northern and western parts of the TCMA but in the central, southern, and eastern areas it is largely buried beneath one or more bedrock units, including where it is confined by the lower Oneota aquitard. The linear fit for all CJDN data is
but the low R2 value indicates this will not provide very accurate estimates of chloride from SC.
Following the same process as for the OPDC, we see that 58% of CJDN measurements are above the generalized background and focusing only on the elevated chloride measurements yields a relation of
and the same data subpopulations yield:
In Figure 7, we see these trends and their associated confidence intervals. Note that the trend for the >15 m of clay points (blue) was not plotted since its R2 is less than 0.5. Distinct from the OPDC case, we see that the trend and confidence interval of the measurements from wells with less clay and no overlying bedrock (red) can be distinguished from the other trends. For wells in that context, we can now use this more robust transfer function to estimate chloride concentration from SC. This trend and the elevated trend will both dramatically improve chloride estimates compared to the bulk CJDN relation in Eq. 10. In those cases, SC actually can be a good proxy for chloride concentration even though the same cannot be said for an arbitrary CJDN well.
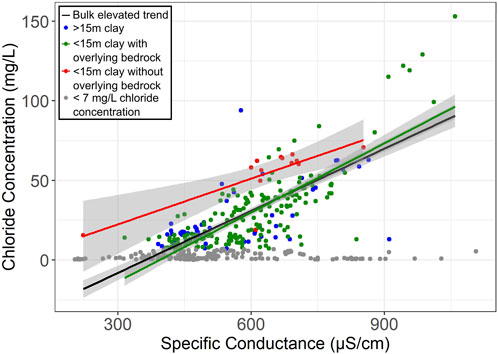
FIGURE 7. SC vs. chloride concentration for the CJDN unit. Grey points are below the estimated background level of 7 mg/L. The black line is the trend of all points above the background threshold. Blue points are from wells overlain by >15 m of Quaternary clay. Red points are from wells with <15 m of overlying clay where CJDN is the uppermost bedrock. Green points denote wells were the CJDN is overlain by one or more bedrock aquitards and there is <15 m of overlying clay. The trend for the blue points is not plotted because it does not reach an R2 of 0.5. Only the red trend is distinguishable from the other subpopulations.
Relative Changes Over Time
Even when there is not a robust transfer function between SC and chloride concentration, relative changes in SC measurements can illuminate how chloride concentrations evolve in groundwater. Because of chloride’s strong impact on SC, changes in chloride concentration tend to be readily apparent in SC time series data. For example, there are 47 wells in the data set with 8 or more paired measurements over the study period. Figure 8 presents the time series data for chloride (a) and SC (b) in one of these wells screened in the CJDN (well #121063). In the figure, it is readily apparent that chloride concentrations and SC are changing in this well following the same general pattern which is characteristic of the large majority of the wells with multiple measurements. A single SC sensor in such a well could provide continuous, if semiquantitative, monitoring of chloride changes.
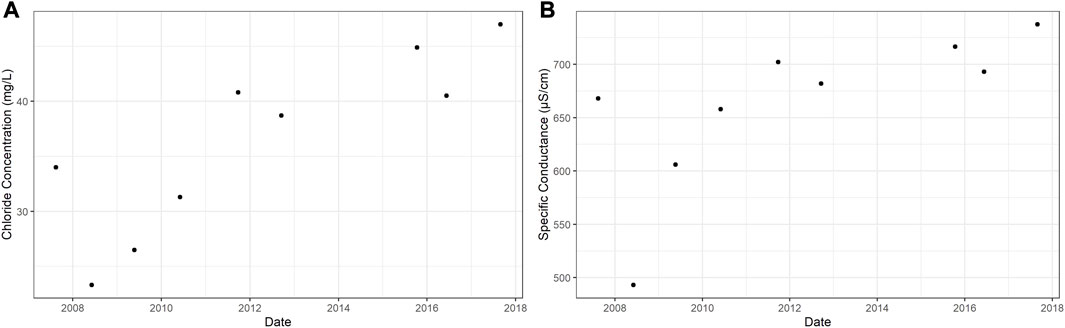
FIGURE 8. Chloride concentration (A) and specific conductance (B) from well #121063 which is screened in the Jordan Sandstone aquifer (CJDN).
We can also make use of relative changes in SC over time to better understand how chloride-enriched recharge is reaching deeper aquifers such as the CJDN. The quantity of rock salt used for road deicing in Minnesota began to increase dramatically in the mid twentieth century, rising ∼10 fold between 1955 and 1965 (Sander et al., 2007; Novotny et al., 2008). Given its strong connectivity to the land surface the QWTA would have received water with elevated chloride concentrations very soon after, and today we see that this unit is permeated with high-chloride waters (McDaris et al., 2022). However, more deeply buried aquifers such as the CJDN remain much more variably impacted by chloride-enriched recharge. Research using residence time indicators such as tritium show that the CJDN has zones of groundwater with pre-1953 mean residence times alongside zones of more recent and mixed waters (post-1953) (Berg, 2016; Berg, 2019; Berg, 2021). A more precise mean residence time of these recent waters has been estimated at approximately 40–50 years using a variety of tracers (DCEM, 2003; Crawford and Lee, 2015; Demuth and Scott, 2020) which means that our data set spans the period during which this anthropogenic chloride likely began to reach the CJDN.
To look for this change in water chemistry, we binned the CJDN data by decade of sample date and computed a linear best fit between SC and chloride concentration in each bin. Only two decades yielded statistically significant trends above the 90% confidence level: the 1970s and the 2010s (Figure 9).
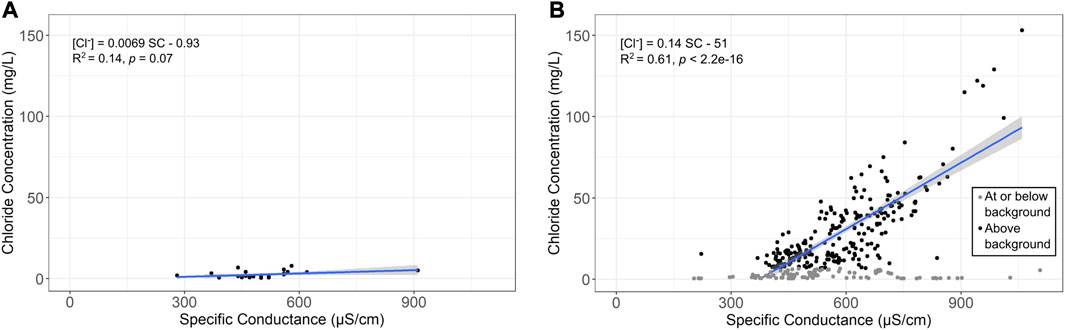
FIGURE 9. SC versus chloride concentration plots of data from the Jordan Sandstone aquifer collected in the 1970s (A) and 2010s (B). For the CJDN, we estimate the natural background chloride concentrations as equal to the mean of the 1970s data plus twice the standard deviation. In (B), point with chloride concentrations at or below that level are plotted in gray and are excluded from the linear fit displayed in blue. Black points in (B) have chloride concentrations higher than the natural background. The trend displayed corresponds to the measurements above the background. Confidence intervals for each regression are shaded grey.
The 1970s data shows almost no correlation between the two factors, and very low chloride concentrations (Figure 9A). With a mean residence time of 40–50 years, CJDN chloride concentrations in the 1970s would not yet have been affected by increased salt use at the surface, and they therefore can be used to directly infer a natural background concentration for this unit. Calculating two standard deviations above the mean of the 1970s data yields a background estimate of 6.7 mg/L which confirms previous research (Kroening and Ferrey, 2013; McDaris et al., 2022) and is consistent with the estimated background presented earlier in this work. By the 2010s, surface waters carrying deicing chloride would have had time to permeate into this unit (Figure 9B). While there are still many wells in which chloride concentrations are low and do not contribute appreciably to SC (gray points), there is a new population of measurements with chloride concentrations elevated well above the natural background (black points) with a handful more than halfway to the chronic water quality threshold of 230 mg/L. The trend associated with these elevated concentrations has nearly the same slope as the overlying OPDC unit (Eq. 5) which illustrates the strengthening connection to shallower waters. (Data and fit statistics are shown for all four decades in Supplementary Figure S1)
It is striking how similar Eq. 15 is to Eq. 14. Eq. 14 was derived from a spatial, hydrogeologic context perspective while Eq. 15 was derived from data constrained by an interval of time. Based on this similarity, we would infer that CJDN wells which had elevated chloride concentrations in the 2010s are likely to be in areas where there is <15 m of clay in the overlying Quaternary deposits. This is borne out when we plot the data based on clay thickness (Figure 10). Combining the temporal and spatial approaches can bring more insights than either approach alone.
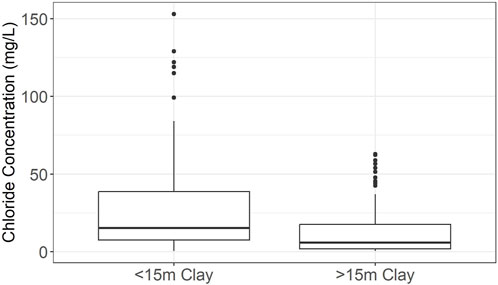
FIGURE 10. Boxplot of chloride concentrations in samples collected in the 2010s from CJDN wells with either more or less than 15 m of clay in overlying deposits.
Conclusion
The use of rock salt for winter deicing will continue unless a viable alternative is developed, which means that chloride will continue to build up in lakes, streams, and groundwater aquifers until the amount leaving the region via rivers is in balance with the amount being added to the system. Mitigating this accumulation relies on understanding how much chloride is being introduced into each of these reservoirs as well as the locations of geologic features that could provide fast pathways to deep aquifers. This level of insight will require more and more frequent data about how chloride concentrations are changing in aquifers than is currently available.
Specific conductance is routinely employed as a proxy for chloride concentration in surface water studies and some groundwater studies. In this work, we have used extensive historical data to examine groundwater in an urban setting where issues with salinity are driven primarily by anthropogenic activity. We have parsed out different hydrogeologic settings within individual aquifers across this multi-aquifer system to evaluate where the SC-chloride proxy is quantitatively robust and have shown that qualitative change over time also holds value.
Our findings reinforce the need to develop a sentinel network of SC sensors in wells across the TCMA gathering continuous measurements at multiple levels in the aquifer system. Data from these sensors can provide a high-resolution view of chloride movement, sub-annual variations, and feedback on the effects of changes in management practices.
Data Availability Statement
Publicly available datasets were analyzed in this study. This data can be found here: https://conservancy.umn.edu/handle/11299/225351 Data Repository for the University of Minnesota.
Author Contributions
Study conception and design: JM and JF; data collection: JM; analysis and interpretation of results: JM, JF, JL, and AR; draft manuscript preparation: JM. All authors contributed to the article and approved the submitted version.
Funding
Partial support for the graduate work of JM has been provided by the families of Subir Banerjee, Richard Clarence Dennis, and Harold M. Mooney through fellowships awarded by the Department of Earth and Environmental Sciences (2020, 2021, and 2022 respectively), as well as the Gill Gabanski Scholarship (2020) from the Minnesota Groundwater Association.
Conflict of Interest
The authors declare that the research was conducted in the absence of any commercial or financial relationships that could be construed as a potential conflict of interest.
Publisher’s Note
All claims expressed in this article are solely those of the authors and do not necessarily represent those of their affiliated organizations, or those of the publisher, the editors and the reviewers. Any product that may be evaluated in this article, or claim that may be made by its manufacturer, is not guaranteed or endorsed by the publisher.
Acknowledgments
We thank two reviewers for their comments, which improved the quality of this paper, and Jack Longman for managing the peer review process. The full data set for this research is available via this in-text data citation reference: McDaris et al. (2021). The University of Minnesota is built on the ancestral lands of the Wahpekute band that was ceded to the United States by the Treaty of Traverse des Sioux in July of 1851, in an agreement that was not paid in full and whose underlying aim was the dissolution of the Dakota culture. The University has also benefited from Chippewa and Dakota (Mdewakanton, Wahpekuta, Wahpeton and Sisseton Bands) land ceded by treaty and given to the University of Minnesota via the Morrill Act. Due to its land-grant status, the infrastructure, financial foundations, and faculty, students, and staff at the University of Minnesota all continue to benefit directly from these ceded lands, and we wish to acknowledge this support in our research.
Supplementary Material
The Supplementary Material for this article can be found online at: https://www.escubed.org/articles/10.3389/esss.2023.10084/full#supplementary-material
References
Abanyeh, H. Z., Nazemi, A. H., Neyshabori, M. R., Mohammadi, K., and Majzoobi, G. H. (2005). Chloride Estimation in Ground Water From Electrical Conductivity Measurement. J. Agric. Sci. 11 (1), 110–114. Available at: http://tarimbilimleri.agri.ankara.edu.tr/2005/111_1/makale20.pdf.
Alexander, S. C., and Alexander, E. C. (1989). Residence Times of Minnesota Groundwaters. J. Minn. Acad. Sci. 55 (1), 48–52.
Anderson, F. S., Levine, J., and Whitaker, T. (2020). Dating a Martian Meteorite With 20 Myr Precision Using a Prototype In-Situ Dating Instrument. Planet. Space Sci. 191, 105007. doi:10.1016/j.pss.2020.105007
Atekwana, E. A., Atekwanaa, E. A., Roweb, R. S., Werkema, D. D., and Legall, F. D. (2004). The Relationship of Total Dissolved Solids Measurements to Bulk Electrical Conductivity in an Aquifer Contaminated With Hydrocarbon. J. Appl. Geophys. 56, 281–294. doi:10.1016/s0926-9851(04)00057-6
Aupiais, J. (2011). Electrophoretic Mobilities of the Isotopes of Chloride and Bromide Ions in Aqueous Solution at 25 °C and Infinite Dilution. J. Solut. Chem. 40, 1629–1644. article 1629. doi:10.1007/s10953-011-9734-y
Berg, J. A. (2016). Geologic Atlas of Anoka County, Minnesota (Part B): Minnesota Department of Natural Resources, County Atlas Series C-27, Report and pls. St. Paul, MN: MN Department of Natural Resources. 7–9.
Berg, J. A. (2019). Groundwater Atlas of Washington County, Minnesota: Minnesota Department of Natural Resources, County Atlas Series C-39, Part B, Report and Plates. 7–9. St. Paul, MN: MN Department of Natural Resources.
Berg, J. A. (2021). Groundwater Atlas of Hennepin County, Minnesota: Minnesota Department of Natural Resources. County Atlas Series C-45, Part B, Report, 3 pls., GIS Files. St. Paul, MN: MN Department of Natural Resources.
Chang, C., Sommerfeldt, T. C., Carefoot, J. M., and Schaalje, C. B. (1983). Relationships of Electrical Conductivity With Total Dissolved Salts and Cation Concentration of Sulfate Dominant Soil Extracts. Can. J. Soil Sci. 63, 79–86. doi:10.4141/cjss83-008
Chen, M., Daroub, S. H., Lang, T. A., and Diaz, O. A. (2006). Specific Conductance and Ionic Characteristics of Farm Canals in the Everglades Agricultural Area. J. Environ. Qual. 35 (1), 141–150. doi:10.2134/jeq2005.0079
Cormier, S. M., Suter, G. W., Yuan, L. L., and Zheng, L. (2011). A Field-Based Aquatic Life Benchmark for Conductivity in Central Appalachian Streams. United States: United States Environmental Protection Agency. EPA/600/R-10/023F.
Cox, M. H., Su, G. W., and Constantz, J. (2007). Heat, Chloride, and Specific Conductance as Ground Water Tracers Near Streams. Groundwater 45 (2), 187–195. doi:10.1111/j.1745-6584.2006.00276.x
Crawford, M., and Lee, T. (2015). “Using Nitrate, Chloride, Sodium, and Sulfate to Calculate Groundwater Age,” in 2015. Sinkholes and the Engineering and Environmental Impacts of Karst: Proceedings of the Fourteenth Multidisciplinary Conference, October 5-9, Rochester, Minnesota: NCKRI Symposium 5. Carlsbad. Editors D. H. Doctor, L. Land, and J. B. Stephenson (New Mexico: National Cave and Karst Research Institute). Available at: https://digitalcommons.usf.edu/cgi/viewcontent.cgi?article=1073&context=sinkhole_2015.
Dakota County Environmental Management (2003). Hasting Area Nitrate Study – Final Report. Dakota County, MN: Dakota. Available at: https://www.co.dakota.mn.us/Environment/WaterResources/WellsDrinkingWater/Pages/hastings-area-nitrate-study.aspx.
Demuth, V., and Scott, S. (2020). Ambient Groundwater Quality Survey 1999-2019. Dakota County Environmental Resources Department. Dakota County, MN: Dakota. Available at: https://www.co.dakota.mn.us/Environment/WaterResources/WellsDrinkingWater/Pages/ambient-groundwater-quality-study.aspx.
Deverel, S. J., and Fujii, R. (1988). Processes Affecting the Distribution of Selenium in Shallow Groundwater of Agricultural Areas, Western San Joaquin Valley, California. Water Resour. Res. 24 (4), 516–524. doi:10.1029/WR024i004p00516
Diamantis, J. B., and Petalas, C. P. (1989). Seawater Intrusion Into Coastal Aquifers of Thrace and its Impact on the Environment. Toxicol. Environ. Chem. 20-21 (1), 291–305. doi:10.1080/02772248909357389
Fernald, A. G., and Guldan, S. J. (2006). Surface Water–Groundwater Interactions Between Irrigation Ditches, Alluvial Aquifers, and Streams. Rev. Fish. Sci. 14 (1-2), 79–89. doi:10.1080/10641260500341320
Fisher, B., and Feinberg, J. M. (2019). Formation Pathways for Iron Oxide Minerals and Geochemical Conditions for Phosphate Retention in Iron Enhanced Sand Filters. St. Paul, MN: UMN Water Resources Center. Retrieved from the University of Minnesota Digital Conservancy. Available at: https://hdl.handle.net/11299/216623.
Fondriest Environmental, Inc (2014). Conductivity, Salinity and Total Dissolved Solids. Fundamentals of Environmental Measurements. Available at: https://www.fondriest.com/environmental-measurements/parameters/water-quality/conductivity-salinity-tds/ (Accessed February, 2023).
Granato, G. E., DeSimone, L. A., Barbaro, J. R., and Jeznach, L. C. (2015). Methods for Evaluating Potential Sources of Chloride in Surface Waters and Groundwaters of the Conterminous United States. Virginia: U.S. Geological Survey Open-File Report 2015-1080. Available at: https://pubs.usgs.gov/of/2015/1080/ofr20151080.pdf.
Hamilton, P. A., and Helsel, D. R. (1995). Effects of Agriculture on Ground-Water Quality in Five Regions of the United States. Groundwater 33 (2), 217–226. doi:10.1111/j.1745-6584.1995.tb00276.x
Hayashi, M. (2004). Temperature-Electrical Conductivity Relation of Water for Environmental Monitoring and Geophysical Data Inversion. Environ. Monit. Assess. 96, 119–128. doi:10.1023/b:emas.0000031719.83065.68
Hem, J. D. (1989). Study and Interpretation of the Chemical Characteristics of Natural Water. Washington, D.C: U.S. Geological Survey, Water Supply Paper 2254.
Kroening, S., and Ferrey, M. (2013). The Condition of Minnesota’s Groundwater, 2007-2011. St. Paul, MN: Minnesota Pollution Control Agency.
Lee, J., Lee, G., and Song, S. (2007). An Interpretation of Changes in Groundwater Level and Electrical Conductivity in Monitoring Wells in Jeiu Island. J. Korean Earth Sci. Soc. 28 (7), 925–935. doi:10.5467/jkess.2007.28.7.925
Lee, S. H., and Rasaiah, J. C. (1996). Molecular Dynamics Simulation of Ion Mobility. 2. Alkali Metal and Halide Ions Using the SPC/E Model for Water at 25 °C. J. Phys. Chem. 100, 1420–1425. doi:10.1021/jp953050c
McDaris, J. R., Feinberg, J. M., Runkel, A. C., Levine, J., Alexander, E. C., and Kasahara, S. (2022). Documentation and Prediction of Increasing Groundwater Chloride in the Twin Cities, Minnesota. Groundwater 60 (6), 837–850. doi:10.1111/gwat.13227
McDaris, J. R., Feinberg, J. M., Runkel, A., Levine, J., Kasahara, S., and Alexander, E. C. (2021). “Twin Cities Metropolitan Area Groundwater Chloride Data, 1965-2021,” in Data Repository for the University of Minnesota. Available at: https://hdl.handle.net/11299/225351 (Accessed November, 23 2021).
Minnesota Department of Health (2023). Minnesota Well Index. Available at: https://www.health.state.mn.us/communities/environment/water/mwi/index.html (Accessed January, 2023).
Minnesota Department of Health (2016). Southeast Minnesota Domestic Well Network 2016 Data Report. Available at: https://www.health.state.mn.us/communities/environment/water/docs/swp/no3report.pdf (Accessed March, 2023).
Minnesota Geological Survey (2023). Minnesota County Well Index Aquifer Unit Codes. Available at: http://mgsweb2.mngs.umn.edu/cwi_doc/aquifer.asp (Accessed April 2023).
Minnesota Pollution Control Agency (1999). Baseline Water Quality of Minnesota’s Principal Aquifers – Region 6, Twin Cities Metropolitan Area. Available at: https://www.pca.state.mn.us/sites/default/files/metro-rpt.pdf (Accessed December, 2022).
Minnesota Pollution Control Agency (2016). Twin Cities Metropolitan Area Chloride Management Plan. MPCA Report Wq-Iw11-06ff. Available at: https://www.pca.state.mn.us/sites/default/files/wq-iw11-06ff.pdf (Accessed January, 2023).
Moore, J., Fanelli, R. M., and Sekellick, A. J. (2020). High-Frequency Data Reveal Deicing Salts Drive Elevated Specific Conductance and Chloride Along With Pervasive and Frequent Exceedances of the U.S. Environmental Protection Agency Aquatic Life Criteria for Chloride in Urban Streams. Environ. Sci. Technol. 54, 778–789. doi:10.1021/acs.est.9b04316
Novotny, E. V., Murphy, D., and Stefan, H. G. (2008). Increase of Urban Lake Salinity by Road Deicing Salt. Sci. Total Environ. 406, 131–144. doi:10.1016/j.scitotenv.2008.07.037
Overbo, A., Heger, S., Kyser, S., Asleson, B., and Gulliver, J. (2019). Chloride Contributions From Water Softeners and Other Domestic, Commercial, Industrial, and Agricultural Sources to Minnesota Waters. Report to the Minnesota Water Quality Association. Available at: http://www.mwqa.com/wp-content/uploads/2019/06/U-of-M-Chloride-Study-2019.pdf (Accessed January, 2023).
Panno, S. V., Kelly, W. R., Weibel, C. P., Krapac, I. G., and Sargent, S. L. (2003). Water Quality and Agrichemical Loading in Two Groundwater Basins of Illinois' Sinkhole Plain. Champaign, IL: Illinois State Geological Survey EG 156.
Rasmussen, T. J., Ziegler, A. C., and Rasmussen, P. P. (2005). Estimation of Constituent Concentrations, Densities, Loads, and Yields in Lower Kansas River, Northeast Kansas, Using Regression Models and Continuous Water-Quality Monitoring, January 2000 through December 2003. Reston, Virginia: U.S. Geological Survey. Scientific Investigations Report 2005–5165, 126. doi:10.3133/sir20055165
Roehl, E. A., Daamen, R. C., and Cook, J. B. (2013). Estimating Seawater Intrusion Impacts on Coastal Intakes as a Result of Climate Change. J. Am. Water Works Assoc. 105 (11), E642–E650. doi:10.5942/jawwa.2013.105.0131
Ross, L. (2013). Groundwater Conditions in Twin Cities Aquifers: Beyond the Prairie du Chien-Jordan Aquifer. St. Paul, MN: Presentation to the Metropolitan Area Water Supply Advisory Committee (Metropolitan Council. Available at: https://bit.ly/2QqS1OS (Accessed October, 2023).
Runkel, A. C., Mossler, J. H., Tipping, R. G., and Bauer, E. J. (2006). A Hydrogeologic and Mapping Investigation of the St. Lawrence Formation in the Twin Cities Metropolitan Area. University of Minnesota. Retrieved from the University of Minnesota Digital Conservancy. Available at: http://hdl.handle.net/11299/108892 (Accessed April, 2023).
Runkel, A. C., Tipping, R. G., Alexander, E. C., Green, J. A., Mossler, J. H., and Alexander, S. C. (2003). RI-61 Hydrogeology of the Paleozoic Bedrock in Southeastern Minnesota. St. Paul, MN: Minnesota Geological Survey. Retrieved from the University of Minnesota Digital Conservancy. Available at: https://hdl.handle.net/11299/58813.
Runkel, A. C., Tipping, R. G., Meyer, J. R., Steenberg, J. R., Retzler, A. J., Parker, B. L., et al. (2018). A Multidisciplinary-Based Conceptual Model of a Fractured Sedimentary Bedrock Aquitard: Improved Prediction of Aquitard Integrity. Hydrogeology J. 26 (7), 2133–2159. doi:10.1007/s10040-018-1794-2
Sander, A., Novotny, E., Mohseni, O., and Stefan, H. (2007). Inventory of Road Salt Use in the Minneapolis/St. Paul Metropolitan Area. Minneapolis, MN: University of Minnesota St. Anthony Falls Laboratory. Project Report No. 503. Available at: https://conservancy.umn.edu/handle/11299/115332 (Accessed January, 2023).
Tipping, R. G. (2012). Characterizing Groundwater Flow in the Twin Cities Metropolitan Area, Minnesota: A Chemical and Hydrostratigraphic Approach. Minneapolis, MN: Doctoral dissertation, University of Minnesota, 186.
World Health Organization (2004). Guidelines for Drinking Water Quality. Geneva: World Health Organization.
Yang, G., and Moyer, D. L. (2020). Estimation of Nonlinear Water-Quality Trends in High-Frequency Monitoring Data. Sci. Total Environ. 715, 136686. doi:10.1016/j.scitotenv.2020.136686
Keywords: groundwater, chloride, conductance, continuous monitoring, hydrogeologic context
Citation: McDaris JR, Feinberg JM, Levine J and Runkel AC (2023) Establishing Specific Conductance-Chloride Relationships for Quaternary and Bedrock Aquifers in the Twin Cities Metropolitan Area, Minnesota, United States. Earth Sci. Syst. Soc. 3:10084. doi: 10.3389/esss.2023.10084
Received: 26 April 2023; Accepted: 09 August 2023;
Published: 22 August 2023.
Edited by:
Jack Longman, University of Oldenburg, GermanyReviewed by:
William Howcroft, Deakin University, AustraliaWalt Kelly, University of Illinois at Urbana-Champaign, United States
Copyright © 2023 McDaris, Feinberg, Levine and Runkel. This is an open-access article distributed under the terms of the Creative Commons Attribution License (CC BY). The use, distribution or reproduction in other forums is permitted, provided the original author(s) and the copyright owner(s) are credited and that the original publication in this journal is cited, in accordance with accepted academic practice. No use, distribution or reproduction is permitted which does not comply with these terms.
*Correspondence: John R. McDaris, mcda0030@umn.edu