- 1Geological Survey of Sweden, Lund, Sweden
- 2Geological Survey of Sweden, Uppsala, Sweden
Anthropogenic geology (AG) is the study of geological materials and morphologies created, reshaped, or otherwise modified by humans and human activity. Geologists and geological surveys need to include anthropogenically created geology into their science discipline to sustainably resource classify human-made deposits driven by socioeconomic models rather than natural forces. Concepts coupled to anthropogenic geology like circular economy, geosystem services, secondary resources, etc., are important features for the implementation of AG into sustainable economics and resource handling. Thus, it is important for geological surveys and other public agents to gain deep knowledge into these concepts and how the public agents can support sustainable use of anthropogenic geological features.
Introduction
The purpose of this paper is to discuss and identify what role the public sector, with special emphasis on the geological surveys, plays in the development of the Anthropogenic Geology (AG) discipline. We do this by highlighting the role of anthropogenic geology in our society today and in the future and connect this discipline with the increasing demand for geologic materials and increasing land use.
The current and future forming of the geological landscape is increasingly dominated by human activity and the major forces forming and shaping different geological environments are socioeconomic as well as geological and geomorphological. Generally, the geological material produced by human activities is the combined results of past and present human activities on the site. Humanity’s impact on the geosphere spans from its surface down to depths of several thousand meters and today, humans are the largest geomorphological force on the planet: in 2015, global anthropogenic production of sediments exceeded natural supply by a factor of 24 (Cooper et al., 2018). Already in the 1950s, human activity took over as the main geomorphological driving force compared to natural sediment flux systems. The post-1950 period of the so called “Great Acceleration” is in general characterized by the large-scale, human-induced changes in socioeconomic and biophysical systems (Steffen et al., 2015) and thus, it has been suggested by the Anthropocene Working Group that this point in time should mark the beginning of this stratigraphic epoch called the “Anthropocene” (Zalasiewicz et al., 2017). Also, anthropogenic processes take place on much shorter time scales than many natural processes and can have a much higher and immediate impact on human society. The proposed Anthropocene is also often looked upon from a “global gaze” (Biermann et al., 2016) and less attention is given to regional and local environmental changes that are entangled within global connections such as the global market. For this reason, human activities and human impacts on the planet and its different regions and societies can be considered as a geological and geophysical system. This system is not driven by mainly natural forces, but by socioeconomic forces. Socioeconomics and behavioural economics are areas not normally connected to geosciences, and particularly not from a scientific perspective. But with increasing geological deposits created by human activity, the geological community and especially the governmental agencies, such as geological surveys, need to address these new geological settings (and processes). To characterize anthropogenic resources from artificial ground there is a need to develop the AG discipline with knowledge tools and methods. This includes the ability to develop and correctly assess the results from geophysical methods (e.g., Sandrin et al., 2020; Jonsson et al., 2023), geochemistry (Jonsson et al., 2023) and new sedimentary settings (Dijkstra et al., 2019) of such deposits.
This paper is divided into four parts. First, a brief review of the development of the anthropogenic geology discipline is presented as well as a suggestion by the authors on a common definition of the term. The second part presents a processual model through which anthropogenic geology is created where we present a model based on socioeconomics as the driving force and give examples of the types of geology that are the result of such a process. In the third part we present three cases from Sweden describing different settings of anthropogenic geology. Finally, in the fourth part we discuss the way forward to map, characterize, and classify anthropogenic geology as resources. We use the United Nations Framework Classification—system (UNFC; UNECE, 2018; UNECE, 2019; UNECE, 2021) as a baseline and show how public sector organisations (geological surveys and other agencies) can contribute to the challenges (as identified by Winterstetter et al., 2021) that needs to be addressed to make anthropogenic geological resources sustainably useful for the society.
A Summary of the History, State, and Development of Anthropogenic Geology
Generally geologists have only recently put interest into the study of anthropogenic deposits, although the idea of artificial ground as a significant geology have a long history (e.g., Sherlock, 1922; Butzer, 1971). Butzer (1971 page 4) concluded in his introduction that Pleistocene geologists have until the 1970’s almost ignored the presence of humans and their impact on the environment. Until recently this used to be a field for archaeologists to study through archaeological stratigraphy, thus the concept anthropogenic geology in part, converge these two fields. Artificial ground was first shown on geological maps in the 1960s (cf. Edgeworth, 2014), but it was not until the 1990s this type of mapping became more of a standard, and still in many areas and countries it is not. Investigation of artificial grounds has mainly concerned assessment of stability and contamination of land for development. However, in relation to discussion of the proposed Anthropocene, and with the emergence of an exploding urbanization in recent decades accelerating exploitation of further nature into anthropogenic materials and resources (Zalasiewicz et al., 2008; 2011)—the concept of anthropogenic geology has become a more profound general interest to geoscientists (Price et al., 2011; Ford et al., 2014). Of special interest is the potential use of anthropogenic (i.e., artificial) materials as a renewed raw material that can be utilised as a replacement for the natural material that would otherwise be needed to be exploited.
There does not seem to exist a clear definition on the term “Anthropogenic Geology.” In a broads sense it can be defined as deposits created as a result of human activity. Several researchers divide anthropogenic deposits according to two overlapping processes (Price et al., 2011; Zalasiewicz et al., 2011): 1) the creation of novel sedimentary environments and sediments (artificial ground) and 2) modifications to natural sedimentary environments through processes such as damming, coastal reclamation or straightening of rivers (Syvitski and Kettner, 2011). Common terms referring to anthropogenic geology include anthropogenic deposit(s), anthropogenic resource(s), anthropic rock, secondary resources, technogenic ground, artificial ground, to mention some (McMillan and Powell, 1999; Price et al., 2011; Zalasiewicz et al., 2011; Ford et al., 2014; Peloggia, 2018a). There are also “Anthropogeomorphology” (sensu Haldar and Sapati, 2018) and “Anthropocene palaeontology” (sensu Williams et al., 2019) as well as other terms that are closely linked to the concept/discipline of AG. This only strengthen the need for a common nomenclature that describes the fact that humans today are a geological force and does create geology through human activity, hence the term “anthropogenic geology.” Thus, we propose a definition of anthropogenic geology as:
Anthropogenic geology is the study of geological materials and morphologies created, reshaped, or otherwise modified by humans and human activity.
This definition is partly synonymous to environmental geology which is defined as the interaction of humans with the geological environment (cf Bennett and Doyle, 1997). Anthropogenic geology singles out the geology that is driven by human actions. Environmental geology is a wider definition on the human effect on all geological environments, including what can be considered as a natural setting. Thus, anthropogenic geology can be seen as a subset of Environmental geology. Peloggia (2018b) considers the rock cycle and suggest a new model which incorporate processes and geological materials produced by humanity. This is much in line with some of the suggestions already made by others (Cathcart, 2011; Ford et al., 2014; Underwood, 2001a; Underwood, 2001b). Peloggia (2018b) tries to expand the anthropogenic part of the Cathcart-Underwood rock-cycle and complement this with a box for the unconsolidated materials produced by humanity, including soils, sediments, technogenic materials and others into this scheme. Also, Peloggia (2018b) concludes a need to further develop and improve this scheme. However, putting the anthropogenic geology as a part of the natural rock cycle is not straightforward since the forces that underly the anthropogenic geology differs from the forces of nature that delimits the other parts of the rock cycle. Instead, the anthropogenic geology is better described by a model based primarily on socioeconomics.
The Socioeconomic Geological Model
The driving force of anthropogenic geology lies in the socioeconomic context, i.e., market forces, behavioural aspects and other driving forces behind the fact that humans alter the Earth in a fashion that is not explained by the forces of nature. Thus, anthropogenic geology is driven by market demand and economic development and results in a novel type of geology and geologic landscape. Figure 1 shows a concept map of the anthropogenic geological model (or the socioeconomically driven geological process).
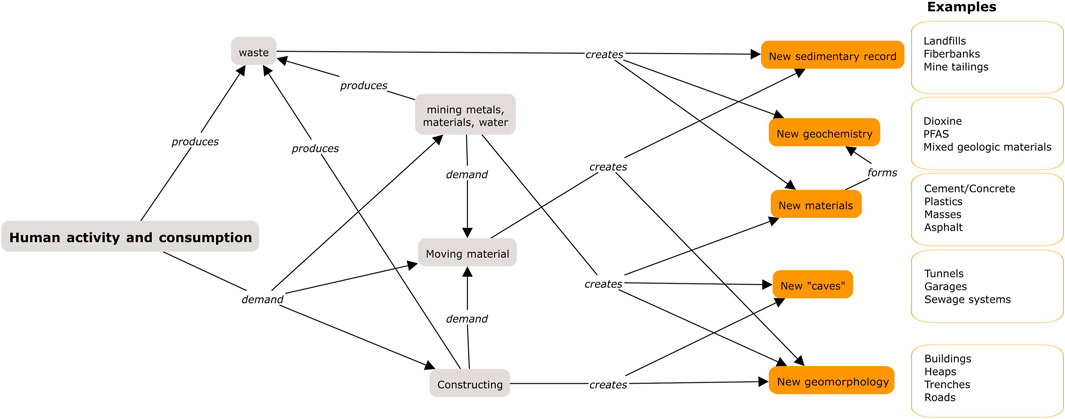
Figure 1. The anthropogenic geological model conceptualized through a socioeconomically driven geological process.
This concept map is not intended as a complete model but emphasizes the societal driving forces of anthropogenic geology, with the human factor as the main contributor. In order to better prospect and map anthropogenically made geological resources the factors and forces driven by the social-geological field is important to understand, including its economic (monetary) systems. Mora (2013) stated:
“As a historical and interpretative science, geology can inform society about interactions in coupled human-environmental systems because our skills and proficiencies allow us to recognize the varying manifestations of phenomena at different spatial and temporal scales.”
The geological discipline is profound for the contribution to the implementation and understanding of the Agenda 2030 and the Global Sustainable Development Goals (SDG:s; Gill, 2017; Stewart and Gill, 2017; Smelror, 2020; Gill and Smith, 2021). Geoscience is in a way the basic pre-requisite for all other human and nature functions and there is today a lack of understanding of this in modern society. This poses a risk that efforts towards preservation and restoration of the nature are in vain, likewise the much-needed inclusion of nature into socioeconomics. Partially, this is due to poor understanding on what impact geology and geological systems have on society, and perhaps even less so for the socioeconomic impact and influence on geology itself.
Anthropogenic geology requires knowledge from not only natural sciences, but a cross-knowledge of natural-, social-, economic-, philosophical- and political sciences. There are several disciplines and concepts, both new and already established, that are relevant to anthropogenic geology. Secondary resources, urban mining, circular economy, as well as different concepts such as geosystem services are all examples of recent developments in socioeconomics where anthropogenic geology play a vital role.
The Concept of Geosystem Services
Geosystem services is a concept that parallels the concept of ecosystem services, and describes the benefits derived from the abiotic part of nature. Lundin Frisk et al. (2022) identified from literature two definitions of geosystem services: geosystem services as underpinned by geodiversity (definition A), and geosystem services as related to services from the subsurface (definition B). Definition A. (sensu Fox et al., 2020 and referring to Gray, 2011), define geosystem services as “all services associated with geodiversity independent of interactions with biotic nature” (Fox et al., 2020). In this definition, geodiversity underpins and specifies the basis for the flow of services stemming from both the biotic and abiotic features of the ecosystem (Gordon et al., 2012; Gordon and Barron, 2013; Gray et al., 2013; Alahuhta et al., 2018). According to Boothroyd and McHenry (2019), the definition of geodiversity is synthesized as “the natural range (diversity) of geological (rocks, minerals, fossils), geomorphological (landforms, topography, physical processes), soil and hydrological features. It includes their assemblages, structures, systems, and contributions to landscapes.” Definition B. is derived from the works by van Ree and van Beukering (2016) and van Ree et al. (2017) that defined geosystem services as “the goods and services that contribute to human wellbeing specifically resulting from the subsurface” (van Ree and van Beukering, 2016). The authors formulated a distinction between the stocks (e.g., mineral resources, stability) and the flows of services (associated with geological, energy and material cycles) stemming from these stocks. The geosystem services are here differentiated from ecosystem services by van Ree and van Beukering (2016) as originating from the deep-seated stocks, rather than from the critical zone where most of the biotic activity takes place where the pedosphere forms a transition zone between the two types of services (van Ree et al., 2017).
As soon as anthropogenic materials are accumulated (or exhumed forming voids) as a part of the ground and subsurface, they will have some form of geosystem function, and subsequently be a part of forming different benefits for humans as geosystem services. Whether or not such services are “true” geosystem services or not may be debated. But one might see the analogue to artificially made parks, grasslands, etc. that are defined as urban Ecosystem Services where “nature” contributes to the human needs in different ways. Thus, AG may well be seen as potential geosystem services, that are custom made by humans. As an example, are different geological functions that are contributing to resilience of a town; infiltration (permeability), storage of water (sewage systems), flood protection (protection walls) etc. These can be naturally occurring or be man-made for that specific purpose.
The concept of geosystem services regardless of chosen definition, will help us define the anthropogenic geology functions and benefits in situ.
Secondary Resources
Of special emphasis is the concept of “Secondary resources.” This concept in geology refers to the mining of a primary resource such as a base metal, and where a secondary resource may be extracted from the residues (waste material) of the beneficiation of the primary resource. Typically, this can be another metal that was not economical at the time of mining, or a material such as silica sand or aggregates.
Secondary resources have grown in attention through several factors:
1. Increased demand in metals due to a long period of economic growth and development as well as increased demand for metals and materials for the energy transition towards more climate friendly energy sources puts pressure on the supply-side. This leads to an increased interest in old waste heaps and tailings from past mining and beneficiation activities (i.e., activities that enhance the content of a mined product like metal in a metal ore), where there might be a potential to re-beneficiate this material through modern and more effective methods and processes (Hallberg and Reginiussen, 2020; Mulenshi et al., 2021).
2. Former metal-contents of possible by-products in the beneficiation processes that where not considered economical is now, due to price and demand increase as well as new technology possible to extract. Several tailings from metal ores are here considered as potential REE-sources (e.g., Moran-Palacios et al., 2019).
3. Tailings are normally considered environmental hazards, and as such need to be remediated. As a part of the remediation process, metals and other materials may be extracted and due to the cost-reduction for the remediation itself, be economical (e.g., Suppes and Heuss-Aßbichler, 2021).
In recent years, the interest in materials and metals derived from secondary resources have increased dramatically and there are ongoing research and development activities all over the world that address different possibilities for the re-use of industrial and mining waste as a source for extraction.
Urban Mining
Urban Mining is a concept that involves activities and processes for recovery of materials from the urban setting. This includes buildings, infrastructure, and waste (cf. Cossu and Williams, 2015). The term “urban mining” was originally used by Jacobs (1969), with the idea of utilizing the cities as the mines of the future. It involves all kinds of materials and entities that may be used as a potential raw material for some form of beneficiation and production. This includes everything from food residues to plastics and building materials as well as different types of waste materials, such as aggregate masses, bricks, and concrete waste, different metal scraps, etc. All these wastes are to be considered as anthropogenic deposits and may be treated as a part of the social-geological system. Sackett (2016) builds on this idea and argues for the elemental consumption cycles of metals, minerals, and carbohydrates (plastics and fuels) are mostly found above ground today, and even more so in the future. He concludes that biogeochemical cycles must produce as much useful resources as they consume for humanity to be truly sustainable to preserve humanity’s place in the biosphere and suggests a more holistic view on mining that address the need to reframe the whole concept [of mining]. Ongondo et al. (2015) introduces a concept of “Distinct Urban Mine” and defines this as “a delimited space within the anthroposphere (i.e., the total human presence throughout the Earth system including culture, technology, built environment, and associated activities; sensu Kuhn and Heckelei, 2010) that has a high concentration of particular products or materials” The concept is drawn as a parallel to primary mines. In contrast, Johansson et al. (2013) argues that the term Urban Mining is inadequate to encompass all the technosphere and suggest the term Technosphere mining instead to more clearly define the stocks and flows derived from the actual technosphere.
Recent estimates emphasises that there is not enough material within the technosphere that is accessible to cover future demands (especially for base and critical metals). For example, less than one-third out of a total of around 60 metals, have more than 50% recycling rate during their lifetime (UNEP et al., 2011). More than half (34 out of 60) of the metals have less than 1 per cent recycling rate. The recovery rate for some critical raw materials (rare earths, indium, gallium, germanium, lithium and beryllium) is less than 1%. Also, estimates show that recycled materials may provide 65% of the energy transition’s need for cobalt, 75% of lithium and up to 90% of nickel by 2050 (Gregoir and van Acker, 2021). Urban Mining needs to be one part of the resource base together with conventional mining as well as extraction from secondary resources (re-mining).
Anthropogenic Geology in the Circular Economy (CE)
In the CE the focus is on minimising the waste fraction by re-entering materials and products before they are considered a waste. Some of these residuals will still end up as entities called waste, scrap, masses, etc. In AG residuals like excavation masses, certain industrial wastes as well as mining waste may be considered as geological deposits and thus allows for a viewpoint that may consider them as potential raw materials to be re-introduced into the CE model (Figure 2).
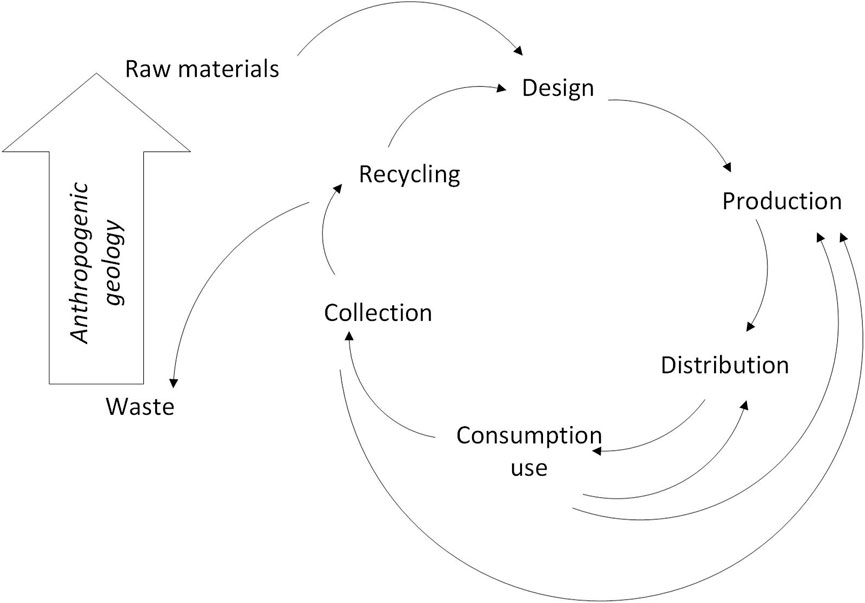
Figure 2. The role of anthropogenic geology for the Circular Economy model. The arrow shows where anthropogenic geology plays a role in closing the ends for the model. Anthropogenic geology as a discipline allows for characterisation of certain waste materials as geologic materials to re-establish such materials as raw material input.
In the circular economic model (cf. European Commission, 2020a; European Commission, 2020b; European Commission, 2020c; Figure 2) as much materials as possible are to stay and re-purpose within the circular chain. This can happen in different stages (Figure 2). The easiest is to reuse the product as it is. Next step is to refurbish, after that step is repair followed by remake and recycle. However, there are losses in the system of material that will end up as waste, and eventually form a anthropogenic deposit. Anthropogenic geology can act as a link back for such materials to re-appear as raw materials for the circular economy again. Thus, AG may assist to connect the end dots of the circular economic system through resource re-classification of waste products and masses, making it even more conceptually sustainable. The concept of CE is an important aspect of the need for AG as a discipline for the present and future.
Anthropogenic geology is vital to obtain correct and optimal re-usage of geological resources. Excavated and unused masses are often considered a cost-bearing and environmental problem and not a potential product of value. A geological context for anthropogenic materials enables the application of methods and models for resource classification to ensure the maximum benefit from such materials. For instance, mineralogical information is important for secondary resource mining, and petrologic, geotechnic, mineralogic and chemical characteristics are important to obtain to assess the re-use and productification of shaft aggregate masses.
There are examples of different CE approaches for AG (Danthurebandara et al., 2015; Kakkos et al., 2020). Kakkos et al. (2020) uses a Life Cycle Assessment (LCA) approach for Urban Mining in construction in Switzerland where the primary resources are seen as a step zero (i.e., Non-circular). Their study estimates the impacts and benefits of conventional versus a circular construction practice applied to various buildings with different parameters and the country-level environmental potential savings that could be achieved through this switch in construction practice. Their results shows on a significant impact on energy and material over conventional methods, strengthening the role for Urban Mining in the circular economy. The role of already present materials and resources such as soils, masses, and ground water remain unclear in these LCAs. Kakkos et al. (2020) illustrates a high need for CE models and methods for assessment of the life-cycle impact of buildings. Development of the anthropogenic geology discipline is key to address parts of construction not defined and included in the LCA-model, such as the ground and the subsurface (sensu Norrman et al., 2021) and derived functions.
Examples of Anthropogenic Geological Systems
Anthropogenic geology is in many ways manifested by the process of urbanization (cf. Ongondo et al., 2015; Chambers et al., 2016; Norrman et al., 2016; Sackett, 2016; Haldar and Sapati, 2018; Hooimeijer and Maring, 2018; Luberti, 2018; Terrington et al., 2018; 2019; Dijkstra et al., 2019; Kakkos et al., 2020; Aldebei and Dombi, 2021). A lot of urban areas today are on artificial ground. Cities, especially very large cities are expanding on landfills and old industrial areas as new ground becomes scarcer due to the expansion itself. Also, new policies on preservation of agricultural and natural environments put further pressure on cities to develop in already exploited areas. However, if we look at where waste is produced and where very large areas of material are deposited, we must look at mining and industry. These types of deposits generate the bulk of waste materials, exceeding urban waste by far. In Sweden in 2020, the mining industry produced approximately 116 Mtonnes of waste, followed by the construction industry in second place that produced a total of 14,2 Mtonnes (SEPA, 2022b). Another important source of waste material is the pulp and paper industry (1,6 Mtonnes in 2020). The total amount of waste generated in Sweden in 2020 was 152 Mtonnes. Thus, the bulk of sedimentary environments produced by humans and anthropogenic activities is found where mines and beneficiation industries are situated, where construction materials and mass deposits are situated, and in the vicinity of paper and pulp industries. From these settings, three cases, all from Sweden, are presented where we further elaborate the geology of such environments.
Fiberbanks–Deposits of Contaminated Industrial Cellulose
Thick deposits of cellulose derived from historical wastewater discharges from the pulp and paper industry are found on shallow sea, lake and river floors, mainly in the northern hemisphere (Poole et al., 1977; Pearson, 1980; Apler et al., 2019; 2020; Snowball et al., 2020). Extensive mapping projects undertaken in Sweden reveals that these anthropogenic derived fiberbanks vary in size from c. 1,000 m2 to 0.5 km2, and the thickness of these deposits generally exceeds 6 m (Apler, 2021). The fiberbanks are today associated with a number of environmental issues, such as pollution of water and benthic fauna (Apler et al., 2020; Dahlberg et al., 2020; 2021), as well as greenhouse gas emissions (Lehoux et al., 2021).
The formation of fiberbanks is closely linked to the development of the pulp and paper industry in the boreal forest region where it has played an important socioeconomic role throughout the 20th and 21st centuries (Kivimaa et al., 2008; Järvinen et al., 2012; Bogdanski, 2014). In the Nordic countries, pulp and paper has been produced from wood raw material since the latter part of the 19th century (Järvinen et al., 2012). A significant increase in pulp and paper production was seen after World War II as the demand for paper products increased in western Europe. This increase correlates with the post-1950 period of the so-called “Great Acceleration,” characterized by large-scale human-induced changes in socioeconomic and biophysical systems (Steffen et al., 2015). At this time, the less developed manufacturing methods resulted in a loss of about 10%–20% of the wooden (cellulose) fibres to the factory wastewater (Norrström, 2015), and the lack of environmental legislation enabled factories to emit suspended particles into adjacent recipient waters where they accumulated. In addition to cellulose and other suspended matter, the wastewater contained process chemicals and metals which were subsequently incorporated into the fiberbanks adsorbed to the cellulose fibres. The cellulose emissions from the pulp and paper industry reached a maximum during the 1960s, after which a regression started due to anti-pollution measures and the closure of many smaller, outdated mills (Jerkeman and Norrström, 2018).
Today, over half a century later, the fiberbanks still remain and there is no estimation of the lifespan over which they will decompose and disappear from the aquatic system. In some places, sedimentation of natural materials on top of fiberbanks occur with potential re-colonialization of lifeforms (Apler et al., 2020). However, barren fiberbank areas are in majority (Norrlin and Josefsson, 2017). A study on the sedimentological archive in a Swedish estuary heavily influenced by historical pulp and paper industry discharges show that metal concentrations diminish over time and that the water quality at the fiberbank sites usually remain below ecotoxicological threshold values (Apler et al., 2019; Apler, 2021). The geological sediment archive from this estuary clearly illustrates the impact of humans with the rise of an industrial era, the introduction of environmental legislation and subsequent aquatic system recovery. Although the established chemostratigraphy demonstrates decreased metal pollution over time it fails to reveal the current hotspots (fiberbanks), which will remain for decades to come. Factors such as shore-line displacement that take place in the northern hemisphere and climate change complicate the long-term assessment of the impact fiberbanks pose on their surroundings. These knowledge gaps in relation to fiberbanks hinder the achievement of national quality objectives and the fulfilment of Agenda 2030 goals.
Currently, fiberbanks are not considered an asset. This is partly due to lack of knowledge on the material characteristics and content of these deposits, and to several unknown and uncertain factors considering environmental effects on the aquatic environment associated to extracting fibres from these banks (Apler et al., 2019; Dahlberg et al., 2020). Pricing of wood for pulp and market demand as well as technical costs for dredging and extraction are other important factors for making the fiberbanks a resource reality. However, fibre extraction as a part of a remediation act is a possibility not tested. Fiberbanks are to be seen as a possible future asset that needs to be mapped and classified to get knowledge of their potential as a pulp-fibre source.
Aggregates and Mass Handling in Urban Areas
Vast amounts of aggregates are needed every year for construction and infrastructure. The yearly consumption of aggregates per person in Sweden is around 10 tonnes. For construction of a normal sized villa ca. 100 tonnes of aggregates are needed, and to build 1 km railroad require ca. 48,000 tonnes of aggregates (SGU, 2022b). The continuous extraction of new geological material from quarried bedrock or natural sand and gravel is not a long-term sustainable solution to our demand for building material, wherefor there is a need to investigate alternative options for the supply of aggregates. Thus, the building sector is particularly prioritized in the new circular economy action plan of the European commission (European commission, 2020a).
The amount of rock aggregates that are being reused in Europe varies significantly between the different countries. Countries that have less access to suitable geology will not be able to produce new rock aggregates to meet the demands. These are countries where, for example, soft sedimentary rocks dominate or there are many conflicting interests for land use. Such countries, including Belgium and the Netherlands, are instead relying on import or reuse of rock aggregate material (British Geological Survey, 2016). In Sweden, less than 5% of the total production of rock aggregates comes from reused material, while the same number in, for example, Belgium is 30% (UEPG, 2023). The low numbers of reused rock aggregates in Sweden could be a result of that crystalline bedrock suitable for aggregate production is widely available over the country, and therefore the incentive of reusing aggregates is less strong. Some other reasons for the low amount of reuse in Sweden could be a lack of ownership and knowledge on how to process and deal with reused rock aggregates, for example, there is not enough spaces to store reused material.
In Sweden, there has been a significant decrease of the natural sand and gravel extraction over the last 25 years, and most aggregates used for construction material are now derived from crushed bedrock (SGU, 2022b). The vast majority (more than 90%) of the material used for aggregate production in Sweden is new material taken directly out of quarries, and a minor part of the bedrock is excavated during large infrastructure projects. The quality of the aggregates depends mostly on their mineralogical, chemical, and physical properties, but also to some extent on the methods used during crushing (Göransson et al., 2018). Therefore, a current key task of the Geological Survey of Sweden (SGU) is to investigate the Swedish geology to determine its rock quality and suitability as aggregates for road, railroad, and concrete. Investigations are particularly focused in areas where there is a large demand, such as in urban areas and in preparation for large infrastructure projects. These types of data can be used for regional material supply planning and the ambition is to optimize the use of excavated material, identify competing interests of the ground and minimize transport during mass handling.
The trend on increasing demand for land for, e.g., urbanisation, nature conservation, natural and material resource excavation, agriculture, forestry as well as culture and recreational purposes gives rise to increase in conflicting interests. The land use pressure is enhanced by climate-change factors that increases the risk for some areas to be inaccessible (heat, sea-level rise, draught, changes in rain-fall patterns, etc.). All such factors limit the availability of natural sand, gravel, and bedrock for aggregate production. Schoning and Mortensen (2021) showed that for the southernmost part of Sweden (Skåne county) the available area for aggregate production is strongly limited and often in areas at a distance from the actual needs. They conclude that new strategies for aggregate material supply needs to be adopted for the area. Thus, promising alternative options for the supply of aggregates to the construction sector may be sought by finding ways to refine the deposits created through the mechanisms of anthropogenic geology. Examples of such deposit resources are aggregate extraction and mass handling during construction work. Crystalline bedrock that is extracted during infrastructure projects such as tunnelling (tunnelling rock, or in Swedish referred to as entreprenadberg) can be of good or poor quality depending on the site’s geological properties. The construction industry branch estimates that between 60–80 Mtonnes of this type of rock is extracted each year during various construction projects. Sometimes it can be reused in the current project or transported for use in nearby projects. However, on many occasions significant amounts of tunnelling rock is not being reused but instead classed as a waste product and taken to a landfill site. This can be due to its properties (i.e., containing elevated amounts of harmful elements), or due to complexity around logistics and laws and legislation. In 2021–2022, the Swedish Environmental Protection Agency (SEPA) led a government assignment to investigate how the society better can take care of and reuse both soil and rock–i.e., shaft masses–that are excavated during infrastructure projects and construction sites (SEPA, 2022a). One of the outcomes of this assignment was a suggestion for the government to make it possible in certain cases to store masses on a site for more than 3 years. This is important for the purpose of reusing the masses in other projects, either as they are or after further processing into new products. One of the goals are to think of the shaft masses more in a way of products rather than just waste material. There is a strong need for an increased knowledge and coordination between different government agencies and industries around material supply planning and mass handling. To optimize the use of crushed new bedrock and strive towards circularity there is a need to classify the anthropogenic deposits, such as shaft masses, and reinstall its material content as valuable products.
More knowledge and research are required on this subject. Deposits from anthropogenic sources need to play an important role in our society. Geological knowledge can be applied on such material and new methods for refining anthropogenic masses may be developed. In the future, geological surveys need to extend their roles from traditional geology to also classify and refine anthropogenic deposits striving towards a circular green economy.
Mining and Ore Processing Waste–Examples From the Swedish Mining Industry
The mining industry produces waste in several steps during the transformation from rock to metal: waste rock from in-mine blasting, waste gravel from crushing and initial separation of the ore, tailing sands from ore processing plants, and slag from metal extraction in foundries and smelters. In each of these steps, the product becomes enriched in the raw material(s) of interest. Any other raw materials, at the time of mining undesired for extraction, thereby become enriched in the different waste products, which makes mining waste and ore processing waste potential value as secondary raw materials (e.g., Blengini et al., 2019). Mine tailings has on and off been of interest for the extraction of additional minerals and/or materials. Extracting from tailings are a question of economic, environmental and social concerns (e.g., Pollmann et al., 2010). Globally there are several attempts to develop methods and models for resource estimations and re-use of tailings (e.g., Parviainen et al., 2020; Araya et al., 2021; Singo and Kramers, 2021; Blannin et al., 2022; 2023; Sarker et al., 2022). This includes methods, characterization on socio economical as well as environmental potentials and issues for both remediation and remining of tailings.
Over more than a thousand years of active mining in Sweden, the total waste rock production is estimated to at least 2 Gt and tailings to more than 1.5 Gt (SGU, 2022a). Approximate estimates of total metal contents in mining waste in Sweden include, e.g., 52 Mt iron, 1.2 Mt zinc, and 1.5 Mt phosphorus (SGU, 2014), i.e., considerable resources if extractable. However, these figures are highly uncertain, due to general unavailability of detailed data. Active mines in Sweden today collect and store information regarding the composition of their waste products, both to comply with environmental legalisation but also for potential future reprocessing. In contrast, data on historical mining are largely lacking, and the little information available is mostly limited to a few elements of interest and excludes trace elements and critical raw materials (CRMs, as defined by the European Commission, 2020b; Grohol and Veeh, 2023).
In recent years, mining waste has gained increased interest as a potential secondary resource for metals and minerals (Huisman et al., 2017), and especially so for CRMs (Blengini et al., 2019). The European Commission recently proposed legislation to force all EU member states to map all their occurrences of mining waste with respect to CRMs (European Commission, 2023), further underlining the importance of mining waste as a secondary resource. As part of the transition into a circular economy, the Swedish government has tasked the SGU with several governmental directives aimed to increase the knowledge about mining waste in Sweden. The early works included exploratory sampling of historical waste rock and tailings with the purpose to identify CRMs in previously unknown locations (Hallberg and Reginiussen, 2018), as well as the development of a systematic sampling method for waste rock heaps (Sädbom and Bäckström, 2018). These efforts were followed by further exploratory sampling, as well as targeted sampling of known CRM-bearing mining waste deposits, expanding the available data on mining waste in Sweden (Hallberg and Reginiussen, 2019; 2020).
In a recently concluded project, the SGU conducted detailed sampling and investigations of historic mining waste deposits selected based on previous data on contents of CRMs and other metals (Jonsson et al., 2023). Systematic sampling of waste rock, tailings, and pyrometallurgical slag was undertaken with the goal to characterize numerous mining waste occurrences and assessing their potential as secondary resources. Drilling and geophysical measurements allowed for analysis and characterization of tailings in three dimensions. Results indicate potentially recoverable concentrations and amounts of several CRMs and other raw materials in waste rock, tailings, and slag; viz. cobalt, phosphorus, rare earth elements, vanadium, tungsten, iron, copper, lead and zinc. However, due to the restricted size of most of the investigated objects, extraction is most likely only economically viable if performed in tandem with primary mining operations. The collected data were further used to develop an application and database for secondary resource potential following the United Nations Framework Classification (UNFC) for Resources to Minerals (UNECE, 2021). The purpose of this database is to highlight the potential of mining wastes as resources for CRMs and other raw materials.
Mining waste may also contain toxic elements and substances, such as arsenic, sulphur, and cadmium, all hazardous for health and the environment if leached into the groundwater, as observed at several locations in Sweden (e.g., Eriksson and Destouni, 1997; Hällström et al., 2020). To this end, SGU is currently responsible for procurement and execution of remediation and nature restoration efforts in areas with hazardous mining wastes. Such ventures mainly aim at encapsulating the toxic materials to prevent leaching to the groundwater, measures that may aggravate future exploration and re-beneficiation of the waste as a secondary mineral resource. Currently, the potential value of mining waste as a secondary mineral resource is generally not considered when planning restoration and remediation endeavours.
Recent studies conclude that lack of data and information act as a barrier, and that establishment of national databases and adoption of classification schemes are needed to bolster the potential extraction of resources from mining waste (Žibret et al., 2020; Suppes and Heuss-Aßbichler, 2021). With a national mission to collect and provide geological data to the society, SGU is the natural host for such databases. Large amounts of data are readily available for compilation, but national campaigns for mapping, sampling and characterisation of mining waste are needed to obtain nationwide coverage of hight quality, detailed data. Application of UNFC to the largest mineral waste deposits in Sweden should emphasize their potential as secondary resources of metals, minerals, and materials. SGU may also promote the development of a more holistic approach to remediation, by which extraction of resources during remediation both provides high-demand raw materials and reduces the total remediation costs for society.
What Role(s) Should the Public Sector Have?
The public sector, and its institutions, are all contributors to shaping the society in different ways. Geological Surveys, Mining Authorities, Departments of infrastructure and housing, and similar public sector agencies are crucial for shaping the society into a sustainable one that complies to the goals set by Agenda 2030 (UN, 2015b), the Kunming-Montreal Global biodiversity framework (UN, 2022), and the Paris agreement (UN, 2015a).
Classification of Anthropogenic Resources
There are a few different classification systems for anthropogenically related geologies, as exemplified by the British Geological Survey (BGS) that uses a classification model for anthropogenic ground based mainly on the settings from which it was formed (Ford et al., 2014). However, to be able to utilize AG as a resource, there is a need to classify AG geology as a resource potential and in connection to such a classification also describe the potential role(s) geological surveys need to develop.
Winterstetter et al. (2021) showed that the United Nations Resource Classification system (UNFC; UNECE, 2018; UNECE, 2019) is highly useable for anthropogenic materials. They identified twelve challenges that needs to be addressed in conjunction to assess and classify anthropogenic geological deposits. Table 1 lists the challenges identified by Winterstetter et al. (2021) and is here complemented by an analysis by the authors on the identified knowledge needed to address each challenge as well as suggestions on what role geological surveys (and similar public sector organisations) needs to have/develop to obtain such knowledge.
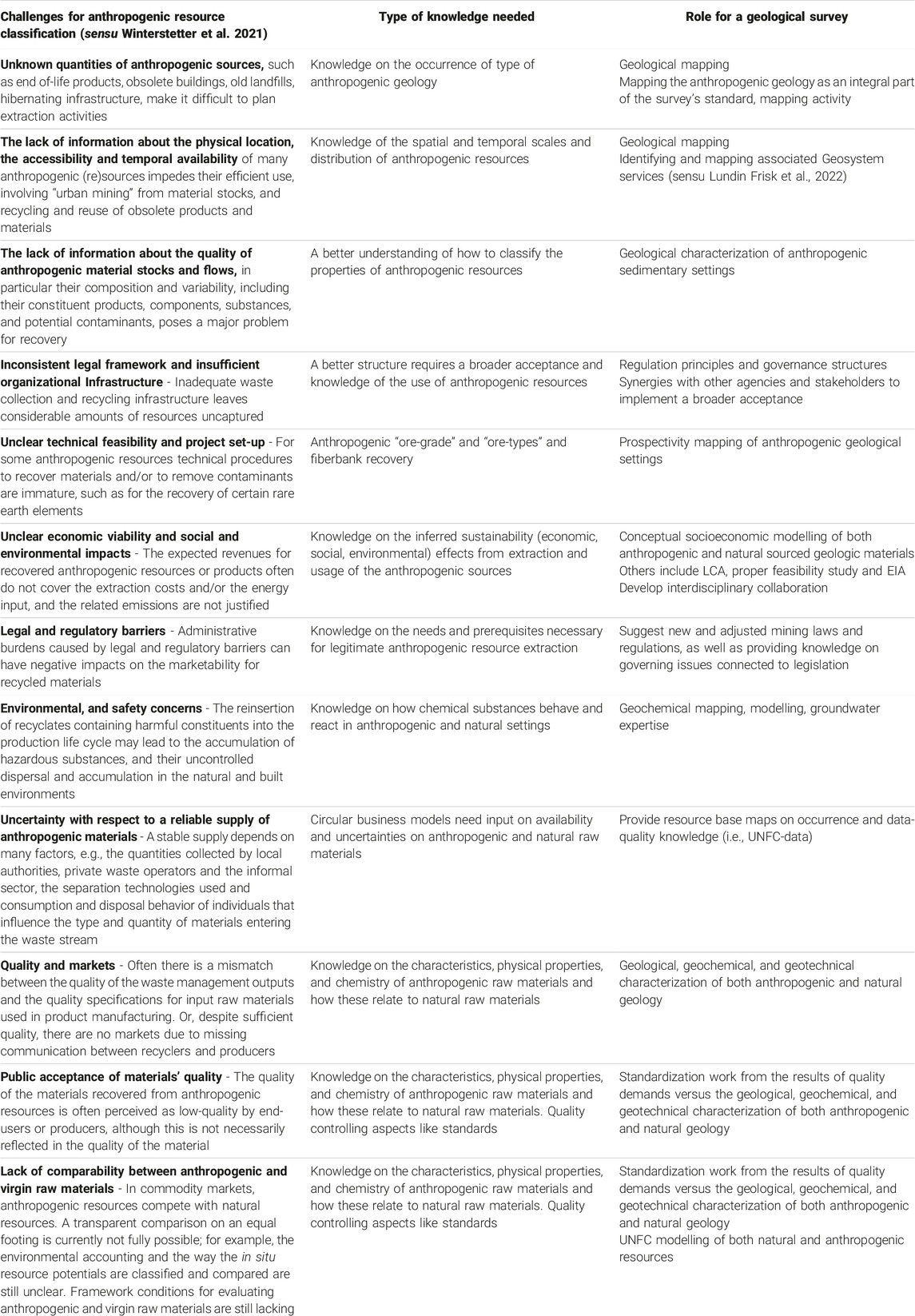
Table 1. Results of an analysis on the twelve challenges as identified by Winterstetter et al. (2021) identifying knowledge needed to address each challenge as well as suggestions on what role geological surveys (and similar public sector organisations) needs to have and develop to obtain such knowledge.
From the analysis of Table 1, four possible areas are apparent where geological surveys and other public sector organisations may have a role. These are mapping, characterization, regulation/governance, and standardization of anthropogenic resources. Mapping is necessary to know where and how anthropogenic deposits and geology occurs. We need to know type as well as spatial and temporal scales. Characterization is needed to understand the resource behaviour (texture, homogeneity, etc.), its genesis (provenance, depositional history) and content (particles, chemistry, petrography). Regulation/governance and standardization are the social and societal connections to the resource uses and sets the desired way to incorporate the resources into sustainable socioeconomic and regulatory policies.
Characterization of Anthropogenic Geology
One role for public sector organisations (i.e., universities and governmental institutions) is to obtain knowledge and develop methodologies to measure and risk-assess the impact on nature and environment from anthropogenically sourced deposits. Knowledge on geochemical and other characteristics are essential for the circular use and handling of such materials. For this reason, there is a need to monitor and test weathering, biogeochemical behaviour and other factors that can make impact on the natural environment, as well as the functionality of the material itself. As pointed out by several authors (e.g., Chambers et al., 2016; Dijkstra et al., 2019), anthropogenically made materials do not have the same maturing processes as naturally derived materials and may contain chemically unstable compounds that are prone to leaching and contamination of the surrounding sedimentary environment. Still, knowledge of anthropogenic material behaviour may then be compared with natural analogues to evaluate the long-term effects of such deposits (Martin et al., 2016).
By using geological methodologies on anthropogenic settings, information comparable to natural geological settings can be obtained. An advantage with such an approach is that presentation and evaluation of the different geologies (natural and anthropogenic) can be made, avoiding some biased knowledge (but also perhaps creating other biases). However, deposited anthropogenic materials (aka technogenic) do not have the same properties and behaviour as natural geologic materials (aka geogenic). Novel methods for characterization are needed since the depositional history of the materials and sediments differ from naturally deposited materials. Geoscientists are suitable for such a task as they are equipped with the ability to work in a forensic systematic way. Geoscientists use shreds of evidence (impacts from past events) to reconstruct geological events and explain their causes. This way of thinking may be useful for the understanding of anthropogenic geology and geoscience.
Anthropogenic Geology and New Concepts
Geological surveys have knowledge on both the natural geological processes as well as societal processes and society’s demands of geological resources. From the position as a bridging role the geological surveys can get a good understanding of how the society creates anthropogenic geology. For instance, a sedimentary geological record is explained by its depositional history from natures geophysical forces (e.g., in petroleum geology and for sand and gravel resources). In natural systems the force of nature creates the geology, and the depositional history is a way to try and explain what conditions created a particular geological geology. Similarly, by knowing the socioeconomic “force of anthroposphere” one can explain the depositional history of a site through correlation in a similar manner to explanation of natural systems. As an example, one can easily attribute new cave systems caused by a demand of metals. We can explain the “depositional history” of such a cave through the mining activity. Even variations in the formation of the cave may be attributed to technologically driven forces. Another example also attributed to the metal demand from society is a tailings dam. The stratigraphy of a tailings dam shows the historical mining activities where changes in chemistry and sediments may correlate to the historical development in technology and needs.
Besides geology and natural science, anthropogenic geology calls for cross disciplinary science where political, social, and economic science are vital parts to fully understand its dynamics. This includes engineering science as well as archaeology and anthropology, cultural and economic geographyand are needed to create explanatory models for different anthropogenic deposits and features. Going back to the three examples presented above, all have very different socioeconomic backgrounds. The fiberbanks are the result of an increasing global need of paper that in turn results in the manufacturing of pulp as a raw material. The waste of such an activity is cellulose fibres. For masses, construction and the human need for buildings and infrastructure drives the development of different masses. And in the third example the same needs for metals that creates new cave systems are also responsible for the creation of fine-grained sand deposits as the waste products of ore beneficiation into tailings deposits. By characterizing and ground truthing such depositional environments with their respective socioeconomic process, the geologist will be able to interpret the different historic anthropogenic deposits without having the full socioeconomic picture for such a deposit. On the other hand, a geologist will also be able to predict the anthropogenic geology that will be created from a certain given socioeconomic process. This knowledge will be vital for understanding the benefits anthropogenic geology can provide as geosystem services as well as determine the “resource potential” from such AG processes in a CE context.
Why Public Sector Organisations?
Do public sector organisations like geological surveys need to collect and interpret anthropogenic geology? Industry and the private sector do in some areas have better knowledge and access to data and information for characterization than geological surveys. For example, the ground engineering industry (geotechnical and civil engineers) encounter the products of human activity regularly and consider it for the design of new structures. Private sector organisations do play a vital role for the development of a sustainable use of anthropogenic geological resources. However, the strength of a public sector organisation lies in its role as a provider of common and accessible information vital for all sectors of a society. Most private sector data is private due to business factors, etc. However, some data and information derived from, for example, ground engineering may be considered as a public domain. For instance, ground data for construction on artificial ground is of high importance for city planning, permitting as well as the public domain. Such data needs to be open, accessible, and trustworthy. This does not mean that the public sector needs to do the investigations, instead they need to have enough knowledge and expertise to be able to know its qualities and to make objective conclusions. This can be described as an interplay between industry, academia, and the public (agencies, politics, etc.; Figure 3) where the role of a state in this context towards industry lies in the development of the framing of the business and discipline through different governance and legislative tools. Also, spatial planning on a societal level is highly needed to develop urban mining as a part of urban development and fabric. Perhaps one of the most important roles for the public sphere is to provide society with information, knowledge and data that is needed to fulfill political goals and for people and business to be able to comply with rules and legislations. Today, a lot of anthropogenic geology is either considered as infilled land, or as a waste and a contaminant. But, as stated in the introduction of this paper, human forces move and deposit materials more than 24 times of what natural forces do, AG will be a dominating geology for human resource needs in the near future if we are to fulfil the needs of humanity sustainably.
Concluding Remarks
This paper and the discussions within can be summarized into the following points:
• Athropogenic geology (AG) is the study of geological materials and morphologies created, reshaped, or otherwise modified by humans and human activity. It is driven by socio-economic processes, like market demand and economic development and results in a novel type of geology and geologic landscape.
• The history of anthropogenic geology can be seen as a document of the human technological development and is often referred as technogenic ground or artificial ground. However, AG can be attributed to a wider geology of new compounds, materials and morphologies.
• Although AG can be seen as a part of environmental geology, it differs contextually as AG is the geology created by human processes while environmental geology is defined as the interaction of humans with the geological environment and has as such a wider scope including human effects on both natural and man-made processes.
• The importance of AG is attributed to its role in sustainability science and can be related to concepts like “Circular Economy,” “Secondary resources,” “Urban Geology” as well as more general concepts of eco-and geosystem services.
• Anthropogenic geology is here exemplified by three different “geologies” from Sweden; fiberbanks off the coast in the Balic Sea, derived from the extensive pulp and paper industry in the region; excavation masses from infrastructure and construction; and secondary resources from present and past mine tailings in the central and northern parts of the country.
• The public sector role for promoting AG as a resource and a discipline includes four major areas: mapping, characterization, regulation/governance, and standardization of anthropogenic resources.
• The strength of a public sector organisation lies in its role as a provider of common and accessible information vital for all sectors of a society, and can be described as an interplay between industry, academia, and the public, where the role of a state in this context towards industry lies in the development of the framing of the business and discipline through different governance and legislative tools.
A conclusion from this paper is that anthropogenic activity is taking over as a major geological force and in order to understand and interpret this “new” geology we also need to understand the complexity that drives its process, its socioeconomic geological model, and its role for sustainability.
Author Contributions
OT and TB contributed to conception and design of the study. OT, PL, CL, and TB made the analysis for Table 1. OT wrote the first draft of the manuscript. OT, PL, AL, AA, and CL wrote sections of the manuscript. All authors contributed to the article and approved the submitted version.
Conflict of Interest
The authors declare that the research was conducted in the absence of any commercial or financial relationships that could be construed as a potential conflict of interest.
Publisher’s Note
All claims expressed in this article are solely those of the authors and do not necessarily represent those of their affiliated organizations, or those of the publisher, the editors and the reviewers. Any product that may be evaluated in this article, or claim that may be made by its manufacturer, is not guaranteed or endorsed by the publisher.
References
Alahuhta, J., Ala-Hulkko, T., Tukiainen, H., Purola, L., Akujarvi, A., Lampinen, R., et al. (2018). The Role of Geodiversity in Providing Ecosystem Services at Broad Scales. Ecol. Indic. 91, 47–56. doi:10.1016/j.ecolind.2018.03.068
Aldebei, F., and Dombi, M. (2021). Mining the Built Environment: Telling the Story of Urban Mining. Buildings 11, 388. doi:10.3390/buildings11090388
Apler, A. (2021). “Contaminated Organic Sediments of Anthropogenic Origin: Impact on Coastal Environments,” in Digital Comprehensive Summaries of Uppsala Dissertations from the Faculty of Science and Technology 1997 (Uppsala: Acta Universitatis Upsaliensis), 76. ISBN 978-91-513-1094-7.
Apler, A., Snowball, I., Frogner-Kockum, P., and Josefsson, S. (2019). Distribution and Dispersal of Metals in Contaminated Fibrous Sediments of Industrial Origin. Chemosphere 215, 470–481. doi:10.1016/j.chemosphere.2018.10.010
Apler, A., Snowball, I., and Josefsson, S. (2020). Dispersal of Cellulose Fibers and Metals From Contaminated Sediments of Industrial Origin in an Estuary. Environ. Pollut. 266, 115182. doi:10.1016/j.envpol.2020.115182
Araya, N., Ramírez, Y., Kraslawski, A., and Cisternas, L. A. (2021). Feasibility of Re-Processing Mine Tailings to Obtain Critical Raw Materials Using Real Options Analysis. J. Environ. Manag. 284, 112060. doi:10.1016/j.jenvman.2021.112060
Bennet, M., and Doyle, P. (1997). Environmental Geology: Geology and the Human Environment. Sussex: John Wiley and Sons Ltd, 501.
Biermann, F., Bai, X., Bondre, N., Broadgate, W., Arthur Chen, C. T., Dube, O. P., et al. (2016). Down to Earth: Contextualizing the Anthropocene. Glob. Environ. Change 39, 341–350. doi:10.1016/j.gloenvcha.2015.11.004
Blannin, R., Frenzel, M., Tolosana-Delgado, R., Büttner, P., and Gutzmer, J. (2023). 3D Geostatistical Modelling of a Tailings Storage Facility: Resource Potential and Environmental Implications. Ore Geol. Rev. 154, 105337. doi:10.1016/j.oregeorev.2023.105337
Blannin, R., Frenzel, M., Tolosana-Delgado, R., and Gutzmer, J. (2022). Towards a Sampling Protocol for the Resource Assessment of Critical Raw Materials in Tailings Storage Facilities. J. Geochem. Explor. 236, 106974. doi:10.1016/j.gexplo.2022.106974
Blengini, G. A., Mathieux, F., Mancini, L., Nyberg, M., and Viegas, H. M. (2019). Recovery of Critical and Other Raw Materials From Mining Waste and Landfills: State of Play on Existing Practices. Luxembourg: Publications Office of the European Union. Science for Policy report by the Joint Research Centre (JRC). EUR 29744 EN.
Bogdanski, B. E. C. (2014). The Rise and Fall of the Canadian Pulp and Paper Sector. For. Chron. 90, 785–793. doi:10.5558/tfc2014-151
Boothroyd, A., and McHenry, M. T. (2019). Old Processes, New Movements: The Inclusion of Geodiversity in Biological and Ecological Discourse. Diversity 11, 216. doi:10.3390/d11110216
British Geological Survey (2016). European Mineral Statistics 2010-14. Available at: https://www2.bgs.ac.uk/mineralsuk/download/ems/EMS20102014.pdf.
Butzer, K. W. (1971). Environment and Archeology: An Ecological Approach to Prehistory. 2nd ed. Chicago: Aldine Publishing Company, 703.
Cathcart, R. B. (2011). Anthropic Rock: A Brief History. Hist. Geo-and Space Sci. 2, 57–74. doi:10.5194/hgss-2-57-2011
Chambers, L. G., Chin, Y. P., Filippelli, G. M., Gardner, C. B., Herndon, E. M., Long, D. T., et al. (2016). Developing the Scientific Framework for Urban Geochemistry. Appl. Geochem. 67, 1–20. doi:10.1016/j.apgeochem.2016.01.005
Cooper, A. H., Brown, T. J., Price, S. J., Ford, J. R., and Waters, C. N. (2018). Humans Are the Most Significant Global Geomorphological Driving Force of the 21st Century. Anthropocene Rev. 5, 222–229. doi:10.1177/2053019618800234
Cossu, R., and Williams, I. D. (2015). Urban Mining: Concepts, Terminology, Challenges. Waste Manag. 45, 1–3. doi:10.1016/j.wasman.2015.09.040
Dahlberg, A. K., Apler, A., Frogner-Kockum, P., Göransson, G., Snowball, I., Wiberg, K., et al. (2021). Dispersal of Persistent Organic Pollutants From Fiber-Contaminated Sediments: Biotic and Abiotic Pathways. J. Soils Sediments 21, 1852–1865. doi:10.1007/s11368-020-02871-1
Dahlberg, A.-K., Apler, A., Vogel, L., Wiberg, K., and Josefsson, S. (2020). Persistent Organic Pollutants in Wood Fiber–Contaminated Sediments From the Baltic Sea. J. Soils Sediments 20, 2471–2483. doi:10.1007/s11368-020-02610-6
Danthurebandara, M., Van Passel, S., Vanderreydt, I., and Van Acker, K. (2015). Assessment of Environmental and Economic Feasibility of Enhanced Landfill Mining. Waste Manag. 45, 434–447. doi:10.1016/j.wasman.2015.01.041
Dijkstra, J. J., Comans, R. N. J., Schokker, J., and van der Meulen, M. J. (2019). The Geological Significance of Novel Anthropogenic Materials: Deposits of Industrial Waste and By-Products. Anthropocene 28, 100229. doi:10.1016/j.ancene.2019.100229
Edgeworth, M. (2014). “The Relationship Between Archaeological Stratigraphy and Artificial Ground and its Significance in the Anthropocene,” in A Stratigraphical Basis for the Anthropocene. Editors C. N. Waters, J. A. Zalasiewicz, M. Williams, M. A. Ellis, and A. M. Snelling (The Geological Society Publishing House, Bath: Geological Society, London, Special Publications), 91–108. doi:10.1144/SP395.3
Eriksson, N., and Destouni, G. (1997). Combined Effects of Dissolution Kinetics, Secondary Mineral Precipitation, and Preferential Flow on Copper Leaching From Mining Waste Rock. Water Resour. Res. 33 (3), 471–483. doi:10.1029/96WR03466
European Commission (2020a). Circular Economy Action Plan: For a Cleaner and More Competitive Europe. Luxembourg: Directorate-General for Communication, Publications Office. Available at: https://data.europa.eu/doi/10.2779/717149.
European Commission (2020b). Critical Raw Materials Resilience: Charting a Path Towards Greater Security and Sustainability. Brussels: European Commission, 23.
European Commission (2020c). Leading the Way to a Global Circular Economy: State of Play and Outlook. Luxembourg: Publications Office. Available at: https://data.europa.eu/doi/10.2779/013167.
European Commission (2023). Proposal for a Regulation of the European Parliament and of the Council Establishing a Framework for Ensuring a Secure and Sustainable Supply of Critical Raw Materials and Amending Regulations (EU) 168/2013, (EU) 2018/858, 2018/1724 and (EU) 2019/1020. COM(2023) 160. Brussels: European Commission.
Ford, J. R., Price, S. J., Cooper, A. H., and Waters, C. N. (2014). “An Assessment of Lithostratigraphy for Anthropogenic Deposits,” in A Stratigraphical Basis for the Anthropocene. Editors C. N. Waters, J. A. Zalasiewicz, M. Williams, M. A. Ellis, and A. M. Snelling (London: Geological Society, London, Special Publications), 55–89. doi:10.1144/SP393.12
Fox, N., Graham, L. J., Eigenbrod, F., Bullock, J. M., and Parks, K. E. (2020). Incorporating Geodiversity in Ecosystem Service Decisions. Ecosyst. People 16 (1), 151–159. doi:10.1080/26395916.2020.1758214
Gill, J., and Smith, M. (2021). Geosciences and the Sustainable Development Goals. Cham: Springer International Publishing, 474. doi:10.1007/978-3-030-38815-7
Gill, J. C. (2017). Geology and the Sustainable Development Goals. Episodes 40, 70–76. doi:10.18814/epiiugs/2017/v40i1/017010
Göransson, M., Wallman, S., Bida, J., Lagerblad, B., Schouenborg, B., Peterson, J., et al. (2018). Kritiska Egenskaper Hos Bergmaterial Och Alternativa Material. MinBaS Innovation Rapport nr 2014-04347.
Gordon, J. E., and Barron, H. F. (2013). The Role of Geodiversity in Delivering Ecosystem Services and Benefits in Scotland. Scott. J. Geol. 49, 41–58. doi:10.1144/sjg2011-465
Gordon, J. E., Barron, H. F., Hansom, J. D., and Thomas, M. F. (2012). Engaging With Geodiversity-Why It Matters. Proc. Geol. Assoc. 123, 1–6. doi:10.1016/j.pgeola.2011.08.002
Gray, M. (2011). Other Nature: Geodiversity and Geosystem Services. Environ. Conserv. 38, 271–274. doi:10.1017/S0376892911000117
Gray, M., Gordon, J. E., and Brown, E. J. (2013). Geodiversity and the Ecosystem Approach: The Contribution of Geoscience in Delivering Integrated Environmental Management. Proc. Geologists’ Assoc. 124 (4), 659–673. doi:10.1016/j.pgeola.2013.01.003
Gregoir, L., and van Acker, K. (2021). Metals for Clean Energy: Pathways to Solving Europe’s Raw Materials Challenge. KU Leuwen på uppdrag av Eurometaux. Available at: https://eurometaux.eu/media/jmxf2qm0/metals-for-clean-energy.pdf.
Grohol, M., and Veeh, C. (2023). Study on the Critical Raw Materials for the EU 2023: Final Report. Brussels: European Commission, 155.
Haldar, A., and Sapati, L. N. (2018). Urban Geo-Forms: Concept and Significance in Anthropogeomorphology. J. Indian Geomorphol. 6, 109–115.
Hallberg, A., and Reginiussen, H. (2018). Slutrapportering Av Regeringsuppdrag Kartläggning Av Innovationskritiska Metaller Och Mineral. RR 2018:05. Sveriges geologiska undersökning, 90.
Hallberg, A., and Reginiussen, H. (2019). Mapping of Innovation-Critical Metals and Minerals. SGU Report 2019:20, Sveriges geologiska undersökning.
Hallberg, A., and Reginiussen, H. (2020). Critical Raw Materials in Ores, Waste Rock and Tailings in Bergslagen. SGU-rapport 2020:38.
Hällström, L., Alakangas, L., and Martinsson, O. (2020). Scheelite Weathering and Tungsten (W) Mobility in Historical Oxidic-Sulfidic Skarn Tailings at Yxsjöberg, Sweden. Environ. Sci. Pollut. Res. 27, 6180–6192. doi:10.1007/s11356-019-07305-1
Hooimeijer, F. L., and Maring, L. (2018). The Significance of the Subsurface in Urban Renewal. J. Urbanism 11, 303–328. doi:10.1080/17549175.2017.1422532
Huisman, J., Leroy, P., Tertre, F., Ljunggren Söderman, M., Chancerel, P., Cassard, D., et al. (2017). Prospecting Secondary Raw Materials in the Urban Mine and Mining Wastes (ProSUM) – Final Report. Brussels, Belgium: European Commission. ISBN: 978-92-808-9060-0 (print), 978-92-808-9061-7 (electronic).
Järvinen, J., Ojala, J., Melander, A., and Lamberg, J.-A. (2012). “The Evolution of Pulp and Paper Industries in Finland, Sweden, and Norway, 1800–2005 – A Comparative Analysis,” in The Evolution of Global Paper Industry 1800–2050. Editors J.-A. Lamberg, J. Ojala, M. Peltoniemi, and T. Särkkä (Dordrecht: Springer Science+Business Media), 19–48.
Jerkeman, P., and Norrström, H. (2018). Historien Om Skogsindustrins Miljöarbete – Vägen Mot Hållbarhet, 312.
Johansson, N., Krook, J., Eklund, M., and Berglund, B. (2013). An Integrated Review of Concepts and Initiatives for Mining the Technosphere: Towards a New Taxonomy. J. Clean. Prod. 55, 35–44. doi:10.1016/j.jclepro.2012.04.007
Jonsson, E., Lewerentz, A., and Persson, L. (2023). Undersökning, Provtagning Och Karaktärisering Av Historiska Gruvavfall. in: SGU & Naturvårdsverket (2023) Rapportering Av Regeringsuppdrag: Hållbar Utvinning Av Metaller Och Mineral Från Sekundära Resurser, 17–35.
Kakkos, E., Heisel, F., Hebel, D. E., and Hischier, R. (2020). Towards Urban Mining – Estimating the Potential Environmental Benefits by Applying an Alternative Construction Practice. A Case Study From Switzerland. Sustainability 12, 5041. doi:10.3390/su12125041
Kivimaa, P., Kautto, P., Hildén, M., and Oksa, J. (2008). What Drives Environmental Innovations in the Nordic Pulp and Paper Industry?: Green Markets and Cleaner Technologies (GMCT), TemaNord NV – 2008:512. Copenhagen: Nordic Council of Ministers. doi:10.6027/TN2008-512
Kuhn, A., and Heckelei, T. (2010). “Anthroposphere,” in Impacts of Global Change on the Hydrological Cycle in West and Northwest Africa. Editors P. Speth, M. Christoph, and B. Diekkrüger (Berlin, Heidelberg: Springer). doi:10.1007/978-3-642-12957-5_8
Lehoux, A. P., Isidorova, A., Collin, F., Koestel, J., Snoeball, I., and Dahlberg, A.-K. (2021). Extreme Gas Production in Anthropogenic Fibrous Sediments: An Overlooked Biogenic Source of Greenhouse Gas Emissions. Sci. Total Environ. 781, 146772. doi:10.1016/j.scitotenv.2021.146772
Luberti, G. M. (2018). Computation of Modern Anthropogenic-Deposit Thicknesses in Urban Areas: A Case Study in Rome, Italy. Anthropocene Rev. 5, 2–27. doi:10.1177/2053019618757252
Lundin Frisk, E., Volchko, Y., Taromi Sandström, O., Söderqvist, T., Ericsson, L. O., Mossmark, F., et al. (2022). The Geosystem Services Concept – What Is It and Can It Support Subsurface Planning? Ecosyst. Serv. 58, 101493. doi:10.1016/j.ecoser.2022.101493
Martin, L. H. J., Leemann, A., Milodowski, A. E., Mäder, U. K., Münch, B., and Giroud, N. (2016). A Natural Cement Analogue Study to Understand the Long-Term Behaviour of Cements in Nuclear Waste Repositories: Maqarin (Jordan). Appl. Geochem. 71, 20–34. doi:10.1016/j.apgeochem.2016.05.009
McMillan, A. A., and Powell, J. H. (1999). BGS Rock Classification Scheme Volume 4. Classification of Artificial (Man-Made) Ground and Natural Superficial Deposits – Applications to Geological Maps and Datasets in the UK. British Geological Survey Research Report RR 99-04.
Mora, G. (2013). The Need for Geologists in Sustainable Development. GSA Today 23 (12), 33–37. doi:10.1130/GSATG185GW.1
Moran-Palacios, H., Ortega-Fernandez, F., Lopez-Castaño, R., and Alvarez-Cabal, J. V. (2019). The Potential of Iron Ore Tailings as Secondary Deposits of Rare Earths. Appl. Sci. 9, 2913. doi:10.3390/app9142913
Mulenshi, J., Gilbricht, S., Chelgani, S. C., and Rosenkranz, J. (2021). Systematic Characterization of Historical Tailings for Possible Remediation and Recovey of Critical Metals and Minerals – The Yxsjöberg Case. J. Geochem. Explor. 226, 10677. doi:10.1016/j.gexplo.2021.106777
Norrlin, J., and Josefsson, S. (2017). Förorenade Fibersediment I Svenska Hav Och Sjöar. SGU report nr 2017:07, Available at: https://resource.sgu.se/dokument/publikation/sgurapport/sgurapport201717rapport/s1707-rapport.pdf.
Norrman, J., Ericsson, L., Nilsson, K., Volchko, Y., Sjöholm, J., Markstedt, A., et al. (2021). Mapping Subsurface Qualities for Planning Purposes: A Pilot Study. IOP Conf. Ser. Earth Environ. Sci. 703, 012011. doi:10.1088/1755-1315/703/1/012011
Norrman, J., Volchko, Y., Hooimeijer, F., Maring, L., Kain, J.-H., Bardos, P., et al. (2016). Integration of the Subsurface and the Surface Sectors for a More Holistic Approach for Sustainable Redevelopment of Urban Brownfields. Sci. Total Environ. 563-564, 879–889. doi:10.1016/j.scitotenv.2016.02.097
Norrström, H. (2015). Miljösituationen I Skogsindustrirecipienter – Produktions-Och Miljöteknisk Utveckling Vid Aktuella Fabriker. Stockholm: IVL Svenska Miljöinstitutet, 85. ÅF roject ID 6034963.
Ongondo, F. O., Williams, I. D., and Whitlock, G. (2015). Distinct Urban Mines: Exploiting Secondary Resources in Unique Anthropogenic Spaces. Waste Manag. 45, 4–9. doi:10.1016/j.wasman.2015.05.026
Parviainen, A., Soto, F., and Caraballo, M. A. (2020). Revalorization of Haveri Au-Cu Mine Tailings (SW Finland) for Potential Reprocessing. J. Geochem. Explor. 218, 106614. doi:10.1016/j.gexplo.2020.106614
Pearson, T. H. (1980). Marine Pollution Effects of Pulp and Paper Industry Wastes. Helgoländer Meeresunters. 33, 340–365. doi:10.1007/BF02414760
Peloggia, A. U. G. (2018a). Geological Classification and Mapping of Technogenic (Artificial) Ground: A Comparative Analysis. São Paulo 39 (2), 1–15. doi:10.5935/0100-929X.20180005
Peloggia, A. U. G. (2018b). O Ciclo das Rochas Do Antropoceno: Inserindo a Agência Humana no Sistema-Terra. Rev. do Inst. Geol. São Paulo 39 (1), 1–13. doi:10.5935/0100-929X.20180001
Pollmann, O., Meyer, S., Blumenstein, O., and van Rensburg, L. (2010). Mine Tailings: Waste or Valuable Resource? Waste Biomass Valorization 1, 451–459. doi:10.1007/s12649-010-9042-6
Poole, N. J., Wildish, D. J., Kristmanson, D. D., and Waldichuk, M. (1977). The Effects of the Pulp and Paper Industry on the Aquatic Environment. C R C Crit. Rev. Environ. Control 8, 153–195. doi:10.1080/10643387709381661
Price, S. J., Ford, J. R., Cooper, A. H., and Neal, C. (2011). “Humans as Major Geological and Geomorphological Agents in the Anthropocene: The Significance of Artificial Ground in Great Britain,” in The Anthropocene: A New Epoch of Geological Time. Editors J. A. Zalasiewicz, M. Williams, A. Haywood, and M. Ellis (Philosophical Transactions of the Royal Society Series A), 1056–1084.
Sackett, P. D. (2016). “Elemental Cycles in the Anthropocene: Mining Aboveground,” in Geoscience for the Public Good and Global Development: Toward a Sustainable Future. Editors G. R. Wessel,, and J. K. Greenberg (Geological Society of America Special Paper), 520, 99–116. doi:10.1130/2016.2520
Sädbom, S., and Bäckström, M. (2018). Sampling of Mining Waste – Historical Background, Experiences, and Suggested Methods. Bergskraft Bergslagen AB, 1–71. BKBAB 18-109 Rep.
Sandrin, A., Maricak, A., Heincke, B. H., Clausen, R. J., Nielsen, J., and Keiding, J. K. (2020). Geophysics for Urban Mining and the First Surveys in Denmark: Rationale, Field Activity and Preliminary Results. GEUS Bull. 44, 5240. doi:10.34194/geusb.v44.5240
Sarker, S. K., Haque, N., Bruckard, W., Bhuiyan, M., and Pramanik, B. P. (2022). Development of a Geospatial Database of Tailing Storage Facilities in Australia Using Satellite Images. Chemosphere 303, 135139. doi:10.1016/j.chemosphere.2022.135139
Schoning, K., and Mortensen, G. M. (2021). Förutsättningar För Hållbar Ballastförsörjning I Skåne Län. SGU-rapport 2021:01, 21pp. Available at: https://resource.sgu.se/dokument/publikation/sgurapport/sgurapport202101rapport/s2101-rapport.pdf.
SEPA (2022a). Hantering Av Schaktmassor Och Annat Naturligt Förekommande Material Som Kan Användas För Anläggningsändamål. Redovisning Av Regeringsuppdrag. Available at: https://www.naturvardsverket.se/49c5a7/contentassets/510ee48eff174af79e11cadc4e8cecf8/skrivelse-uppdrag-om-hantering-av-schaktmassor-m2021-00191.pdf.
SEPA (2022b). Avfall I Sverige 2020. Uppkomst Och Behandling. Rapport 7048, Available at: https://www.naturvardsverket.se/4ac5db/globalassets/media/publikationer-pdf/7000/978-91-620-7048-9.pdf.
SGU (2014). Uppdrag Att Utföra en Kartläggning och Analys av Utvinnings-Och Återvinningspotential för Svenska Metall-Och Mineraltillgångar. Geological Survey of Sweden, 66.
SGU (2022b). Statistics of the Swedish Aggregate Production 2021 (In Swedish). SGU Periodiska Publikationer, 44. Available at: https://resource.sgu.se/dokument/publikation/pp/pp202203rapport/pp2022-3-rapport.pdf.
Sherlock, R. L. (1922). Man as a Geological Agent: An Account of His Actions on Inanimate Nature. London: H. F. and G. Witherby, 372.
Singo, N. K., and Kramers, J. D. (2021). Feasibility of Tailings Retreatment to Unlock Value and Create Environmental Sustainability of the Louis Moore Tailings Dump Near Giyani, South Africa. J. South. Afr. Inst. Min. Metallurgy 121, 361–367. doi:10.17159/2411-9717/1138/2021
Smelror, M. (2020). “Geology for Society in 2058: Some Down-To-Earth Perspectives,” in The Changing Role of Geological Surveys. Editors P. R. Hill, D. Lebel, M. Hitzman, M. Smelror, and H. Thorleifson (London: Geological Society). Special Publications 499. doi:10.1144/SP499-2019-40
Snowball, I., Apler, A., Dahlberg, A.-K., Frogner-Kockum, P., Göransson, G., Hedfors, J., et al. (2020). TREASURE – Targeting Emerging Contaminated Sediments Along the Uplifting Northern Baltic Coast of Sweden for Remediation – En Sammanfattning Av Ett Fyraårigt Forskningsprojekt Om Fiberbankar Inom Forskningsprogrammet TUFFO. SGI, Linköping: Statens geotekniska institut, 125.
Steffen, W., Broadgate, W., Deutsch, L., Gaffney, O., and Ludwig, C. (2015). The Trajectory of the Anthropocene: The Great Acceleration. Anthropocene Rev. 2, 81–98. doi:10.1177/2053019614564785
Stewart, I. S., and Gill, J. C. (2017). Social Geology – Integrating Sustainability Concepts Into Earth Sciences. Proc. Geologists’ Assoc. 128, 165–172. doi:10.1016/j.pgeola.2017.01.002
Suppes, R., and Heuss-Aßbichler, S. (2021). How to Identify Potentials and Barriers of Raw Materials Recovery From Tailings? Part I: A UNFC-Compliant Screening Approach for Site Selection. Resources 10, 26. doi:10.3390/resources10030026
Syvitski, J. P. M., and Kettner, A. (2011). “Sediment Flux in the Anthropocene,” in The Anthropocene: A New Epoch of Geological Time. Editors J. A. Zalasiewicz, M. Williams, A. Haywood, and M. Ellis (Philosophical Transactions of the Royal Society Series A), 957–975.
Terrington, R. L., Silva, E. C. N., Waters, C. N., Smith, H., and Thorpe, S. (2018). Quantifying Anthropogenic Modification of the Shallow Geosphere in Central London, UK. Geomorphology 219, 15–34. doi:10.1016/j.geomorph.2018.07.005
Terrington, R. L., Thorpe, S., Kessler, H., Bidarmaghz, A., Choudhary, R., Yuan, M., et al. (2019). “Making Geology Relevant for Infrastructure and Planning,” in International Conference on Smart Infrastructure and Construction 2019 (ICSIC): Driving Data-Informed Decision-Making. Editors M. J. DeJong, J. M. Schooling, and G. M. B. Viggiani (Leeds: ICE Publishing), 403–409. doi:10.1680/icsic.64669.403
UEPG (2023). Aggregates Europe – UEPG. Available at: https://www.aggregates-europe.eu/ (Accessed December 14, 2023).
UN (2015a). The Paris Agreement. Available at: https://unfccc.int/sites/default/files/english_paris_agreement.pdf.
UN (2015b). Transforming Our World: The 2030 Agenda for Sustainable Development. Available at: https://sdgs.un.org/publications/transforming-our-world-2030-agenda-sustainable-development-17981.
UN (2022). Kunming-Montreal Global Biodiversity Framework. Available at: https://www.cbd.int/doc/c/e6d3/cd1d/daf663719a03902a9b116c34/cop-15-l-25-en.pdf.
Underwood, J. R. (2001a). Anthropic Rocks as a Fourth Basic Class. Environ. Eng. Geoscience 7, 104–110. doi:10.2113/gseegeosci.7.1.104
UNECE (2018). Specifications for the Application of the United Nations Framework Classification for Resources to Anthropogenic Resources. Available at: https://unece.org/fileadmin/DAM/energy/se/pdfs/UNFC/Anthropogenic_Resources/UNFC_Antropogenic_Resource_Specifications.pdf.
UNECE (2019). United Nations Framework Classification for Resources – Update 2019. ECE Energy Series https://unece.org/DAM/energy/se/pdfs/UNFC/publ/UNFC_ES61_Update_2019.pdf.
UNECE (2021). Supplementary Specifications for the Application of the United Nations Framework Classification for Resources to Minerals. Geneva: UNECE.
UNEP Graedel, T. E., Allwood, J., Birat, J.-P., Reck, B. K., Sibley, S. F., Sonnemann, G., et al. (2011). Recycling Rates of Metals – A Status Report. A Report of the Working Group on Global Metal Flows to the International Resource Panel. Available at: https://www.resourcepanel.org/reports/recycling-rates-metals.
van Ree, C. C. D. F., and van Beukering, P. J. H. (2016). Geosystem Services: A Concept in Support of Sustainable Development of the Subsurface. Ecosyst. Serv. 20, 30–36. doi:10.1016/j.ecoser.2016.06.004
van Ree, C. C. D. F., van Beukering, P. J. H., and Boekestijn, J. (2017). Geosystem Services: A Hidden Link in Ecosystem Management. Ecosyst. Serv. 26, 58–69. doi:10.1016/j.ecoser.2017.05.013
Williams, M., Zalasiewicz, J., Waters, C., Himson, S., Summerhayes, C., Barnosky, A., et al. (2019). Anthropocene Palaeontology. - Abstracts, Global Markers of the Anthropocene Workshop (Max-Planck-Institute for the Science of Human History). Berlin: Haus der Kulturen der Welt. 18.-20.02.2019.
Winterstetter, A., Heuss-Assbichler, S., Stegemann, J., Kral, U., Wäger, P., Osmani, M., et al. (2021). The Role of Anthropogenic Resource Classification in Supporting the Transition to a Circular Economy. J. Clean. Prod. 297, 126753. doi:10.1016/j.jclepro.2021.126753
Zalasiewicz, J., Waters, C. N., Summerhayes, C. P., Wolfe, A. P., Barnosky, A. D., Cearreta, A., et al. (2017). The Working Group on the Anthropocene: Summary of Evidence and Interim Recommendations. Anthropocene 19, 55–60. doi:10.1016/j.ancene.2017.09.001
Zalasiewicz, J., Williams, M., Barry, T. L., Coe, A. L., Bown, P. R., Brenchley, P., et al. (2008). Are We Now Living in the Anthropocene? GSA Today 18, 3–8. doi:10.1130/GSAT01802A.1
Zalasiewicz, J., Williams, M., Fortey, R., Smith, A., Barry, T. L., Coe, A. L., et al. (2011). Stratigraphy of the Anthropocene. Philosophical Trans. R. Soc. A 369, 1036–1055. doi:10.1098/rsta2010.0315
Žibret, G., Lemiere, B., Mendez, A.-M., Cormio, C., Sinnett, D., Cleall, P., et al. (2020). National Mineral Waste Databases as an Information Source for Assessing Material Recovery Potential From Mine Waste, Tailings and Metallurgical Waste. Tailings Metallurgical Waste. Minerals 10, 446. doi:10.3390/min10050446
Keywords: anthropogenic geology, resource classification, secondary resources, socioeconomic model, sustainability
Citation: Taromi Sandström O, Lindgren P, Lewerentz A, Apler A, Liljenstolpe C and Bejgarn T (2024) Anthropogenic Geology and the Role of Public Sector Organisations. Earth Sci. Syst. Soc. 4:10095. doi: 10.3389/esss.2024.10095
Received: 11 September 2023; Accepted: 06 March 2024;
Published: 18 March 2024.
Edited by:
Donald John MacAllister, British Geological Survey—The Lyell Centre, United KingdomReviewed by:
Simon Price, AtkinsRéalis, CanadaNicholas Hayman, Oklahoma Geological Survey (OGS), United States
Simon David Turner, University College London, United Kingdom
Copyright © 2024 Taromi Sandström, Lindgren, Lewerentz, Apler, Liljenstolpe and Bejgarn. This is an open-access article distributed under the terms of the Creative Commons Attribution License (CC BY). The use, distribution or reproduction in other forums is permitted, provided the original author(s) and the copyright owner(s) are credited and that the original publication in this journal is cited, in accordance with accepted academic practice. No use, distribution or reproduction is permitted which does not comply with these terms.
*Correspondence: Olof Taromi Sandström, olof.sandstrom@sgu.se